Optical Coherence Tomography Angiography
Ruikang K. Wang, PhD; Qinqin Zhang, PhD; Giovanni Gregori, PhD; and Philip J. Rosenfeld, MD, PhD
Optical coherence tomography (OCT) has become one of the most powerful imaging modalities in routine ophthalmic practice since its first invention in the early 1990s.1–6 Its attributes of noninvasive, noncontact, high-resolution (< 10 μm in tissue), and depth-resolved imaging of the anatomical (structural) information within biological tissue have been the key factors for its success. While promising, traditional structural OCT imaging does not provide functional flow information about the dynamics of blood vessel networks. In response to this limitation, OCT angiography (OCTA) has been developed recently and has moved rapidly from the laboratory to clinical imaging in ophthalmology within a short period of time.
In addition to the advantages of traditional OCT imaging, OCTA has the ability to provide in vivo volumetric visualization of microvascular networks within tissue beds without the need for exogenous contrast agents. In Fourier-domain OCT, the signal output is naturally a complex signal, containing both magnitude (intensity) and phase information after Fourier transformation. Both magnitude and phase information have been explored, either individually or in combination, to develop angiographic methods to identify microvascular networks in living tissue. Numerous OCTA algorithms have been proposed, which can be classified into 3 groups: (1) angiography based on a complex signal containing both magnitude and phase portion, such as optical microangiography (OMAG);7,8 (2) angiography based on the magnitude of the OCT signal, such as speckle variance,9 split-spectrum amplitude-decorrelation angiography (SSADA);10 and (3) angiography based on phase signal alone, such as phase variance.11
OMAG is the leading technique that uses the complex OCT signal instead of operating on the phase or magnitude (intensity) alone.7,8 It has been applied to visualize capillary networks in the retina and choroid with high resolution and high contrast.12,13 OMAG has also demonstrated its clinical utility by imaging a range of retinal diseases and choroidal diseases, including diabetic retinopathy (DR),14,15 macular telangiectasia type 2 (MacTel2),16 and choroidal neovascularization (CNV).17,18 The OMAG images are as good as or better than the images obtained using fluorescein angiography (FA) and indocyanine green angiography (ICGA). This chapter briefly describes its working principle and some promising clinical demonstrations.
Figure 9-1. Schematic of flow detection using OCT-based angiography. Assume there are 5 pixels in the A-scan, of which 3 pixels (1, 2, and 5) are located at the static tissue background, and the other 2 pixels (3 and 4) are located within a functional blood vessel. As moving blood cells pass through vessels, they generate dynamic changes in the OCT signals for pixels 3 and 4, but not for pixels of 1, 2, and 5.
BRIEF PRINCIPLE OF OPTICAL COHERENCE TOMOGRAPHY ANGIOGRAPHY/OPTICAL MICROANGIOGRAPHY
The concept of OCTA is to use the changes in OCT signals caused by moving particles (eg, red blood cells) as the contrast mechanism for imaging functional blood flows. To describe briefly its mechanism, let’s first consider 2 OCT signals: one is captured from a static structural tissue, and the other is obtained from moving particles (eg, red blood cells) in functional blood vessels. It is straightforward that the signal from structural tissue would keep steady and unchanged over time, while the signal from flowing blood changes over time because of blood cells passing through the vessel. To differentiate the moving particles from static tissue, it is usually required for OCT probe to capture the signal at a same position repeatedly to build up a time series of OCT signals. This is achieved by repeated OCT cross-sectional scans (B-scans) at the same location in the current OCTA clinical systems. It would not be difficult to appreciate that the temporal changes in the OCT signal between adjacent scans caused by moving particles can be used to generate the angiographic contrast and allow visualization of the functional microvasculature in OCTA (Figure 9-1).
As illustrated in Figure 9-1, a time series of OCT signals are required to contrast blood flow. To fulfill this requirement, a repeated B-mode scanning protocol is adopted to acquire volumetric datasets for the purpose of extracting vasculature networks, ie, a number of B-scans were repeated at individual spatial steps over the slow axis direction (y-axis). After the acquisition of volumetric OCT datasets, a direct differentiation of complex OCT signals between two repeated B-scans was calculated and averaged for all repetitions at the same location to detect the variation induced by moving particles within patent vessels, and this concept constitutes the backbone of the OMAG algorithm:
Where i is the index of repeated B-scans at each location along the slow axis (which is linear with the time t), x is the fast axis scan position, z is the depth, and N is the number of repeated B-scans in each location. C is the complex OCT signal, which can be written as Aexp(-i∅) where A is the magnitude and Φ is the phase of the OCT signal.
As discussed previously, the essence of the OMAG algorithm is to use the differentiation of adjacent B-scan signals captured at the same tissue location. This differentiation is performed on intact OCT signals (ie, complex signals). By simple mathematical operations, it would be straightforward to show that
Figure 9-2. The scheme depicts the cluster of B-scans used in the scan protocol for the optical microangiography approach that generates the blood flow image.
Where ΔΦ is the phase difference (ie, phase variance) between the complex signals Ci(x,z) and C(i+1)(x,z). The term Ai+1(x,z)2 + Ai(x,z)2 is the summation of OCT intensity signal. The term in the bracket represents the complex decorrelation of the signal Ci(x,z) and Ci+1(x,z). In this case, the OMAG algorithm simply represents a complex decorrelation operation on the time series of OCT signals, but weighted by tissue structural information.
However, if only the magnitude of OCT signal is involved in the differentiation operation, then equation (1) would be reduced to the evaluation of OCT structural signal fluctuation. Since the OCT structural signal fluctuation represents the speckle signal, in this case, the OMAG algorithm can be considered as the approximation of speckle variance evaluation.
IMPLEMENTATION OF THE OPTICAL MICROANGIOGRAPHY ALGORITHM
As discussed previously, to contrast the flow signal, the technique of OCTA requires repeated measurements at the same location. In addition, because the retinal blood vessels are densely packed within tissue, there is a requirement of scan density (ie, spacing between adjacent A-scans), in order for OCTA to properly contrast capillary vessels with an average diameter of about 8 μm so that quantification of networks can be made possible. To fulfill these requirements, an improved scanning protocol was introduced for the OMAG algorithm. For the results presented in this chapter, the scanning protocol is described next.
For the spectral domain OCT (SD-OCT) images, 245 A-scans were sampled in the fast axis (x-axis) that forms a B-scan, covering a lateral distance of ~2.4 mm. In the slow scanning direction, the scan was stepped by 245 positions through a range of ~2.4 mm. At the individual spatial positions, a cluster of scans was performed and defined as a number of repeated B-scans. The cluster of scans consisted of 4 repeated B-scans. Therefore, a total of 980 B-scans were captured in the slow axis (y-axis). In doing so, the spacing between adjacent A-scans was slightly less than 10 μm. We employed this scanning protocol in a prototype of the Cirrus 5000 HD-OCT angiography (Carl Zeiss Meditec Inc) instrument to generate the results presented in this chapter. The prototype system operated on a central wavelength of 840 nm with an A-scan speed of 68,000 A-lines/s. Given these parameters, the time interval between 2 successive B-scans was ~4.5 ms, roughly corresponding to a frame rate of 222 frames/second. Hence, the total time for a single volume acquisition was about ~4.4 s, not including the adjustment time before the data collection.
After the data acquisition, the OMAG algorithm was then applied onto each cluster of scans within the 3-dimensional (3D) dataset for the flow signal extraction. An averaged structural B-scan and OMAG flow B-scan was generated at each location (Figure 9-2).
One issue that needed to be addressed when implementing the OMAG algorithm to image the retinal blood flow was the motion of participants. The human eye is in constant motion caused by involuntary fixational eye movements, presenting a major challenge to generating high-quality and high-fidelity OCTA angiograms. To mitigate this problem of eye motion, the Zeiss HD 5000 SD-OCT system is equipped with a motion-tracking correction technique known as FastTrac. The FastTrac technique uses the laser scanning ophthalmoscope to track eye motion and correct it in real time.
Another feature of the HD 5000 SD-OCT system with FastTrac capability is that wide-field OMAG images can be achieved through a montage scanning protocol. In brief, the system can be programmed to provide M × M cube scans, each located at predefined spatial locations with an overlap of 10% between adjacent cubes. For each cube scan, the same scanning strategy as discussed before was employed. The results of individual cubes were then automatically stitched to generate a wide-field retinal angiogram. With this montaging protocol, a field of view of more than 50 degrees has been demonstrated, comparable to the typical field of view offered by FA or ICGA.19
Figure 9-3. The scheme for macular segmentation of a normal human retina. (A) The segmentation of retinal layers. (B) Color-coded information depicting flow within different segmentation layers. (C) En face color-coded OMAG angiogram of the total retinal vasculature. (D) En face OMAG angiogram of the superficial retinal layer (SRL). (E) En face OMAG angiogram of the deep retinal layer (DRL). (F) En face OMAG angiogram of the outer retinal layer (ORL), which is avascular in a normal human retina. (H) En face OMAG angiogram of the ORL to the choriocapillaris (ORCC). (I) En face OMAG angiogram of the CC. (J) En face OMAG angiogram of the choroidal layer. The image size is 2.4 × 2.4 mm.
After the application of the OMAG algorithm, 2 volumetric datasets resulted in parallel: one is structural (4 B-scans averaged at the same location, thus resulting in a better signal-to-noise ratio compared with conventional OCT scans) and the other represents the OMAG flow signals (3 B-scans averaged at the same location), on which the angiograms of retinal capillaries and the choroidal microvasculature can be achieved.
To enhance the visualization of the microvasculature, a semiautomatic segmentation algorithm has been developed that is based on the intensity difference of the OCT cross-sectional structural images.20 The algorithm is capable of separating the retinal layer into individual depth-resolved layers, with sufficient accuracy so as to generate en face projection angiograms according to the retinal anatomy. Generally, 3 retinal slabs are segmented for the retina layer including the superficial retinal layer (SRL, from the inner limiting membrane to the inner plexiform layer), the deep retinal layer (DRL, from the inner nuclear layer to the outer plexiform layer), and the outer retinal layer (ORL, from the outer nuclear layer to the external limiting membrane). In the choroid, 3 additional layers were segmented including the ORL to the choriocapillaris (ORCC) layer, which is about 8 μm beneath Bruch’s membrane; a slab from 8 μm to 20 μm beneath Bruch’s membrane, which includes the remaining CC and inner choroid; and a slab encompassing the choroidal layers beneath the CC to the boundary of sclera. After segmentation, the maximum intensity projection (MIP) along the depth direction was applied for each individual layer to generate en face angiograms. To better appreciate depth-resolved microvasculature, colors can be coded on different layers, where the SRL is colored red, the DRL green, and the ORL blue. The vasculature from ORCC is coded with purple, the CC is coded with red, and the rest of the choroidal vessel is coded with green.
To illustrate the segmentation, a normal human retina was imaged for the purpose of appreciating the vascular appearance of the different layers (Figure 9-3). Figure 9-3A illustrates a typical cross-sectional structural OCT image overlaid with the segmentation lines. The flow signals overlaid on the structural image with color-coding in different layers are shown in Figure 9-3B. For the individual layers in 3D (color-coding in Figure 9-3C), the MIP OMAG angiograms are presented in Figures 9-3D to 9-3J. Microvascular networks in SRL are shown in Figure 9-3D with large vessels. Diving into the deep layer, a denser capillary plexus is seen (Figure 9-3E). The ORL is an avascular layer and represented by a dark signal on the OMAG angiogram for a normal retina. By extending 8 μm beneath the Bruch’s membrane, the angiogram of the ORCC layer (Figure 9-3H), which includes the retinal pigment epithelium (RPE) layer, shows projection artifacts from the overlying retinal circulation. In a normal individual, the CC layer is formed by a very dense, honeycomb capillary array according to existing histology. Because of the limited spatial resolution of the system employed, the OMAG image generated from the CC shows a uniform signal appearance (Figure 9-3I). While the choroidal layer is ramified with larger vessels, these vessels are not orthogonal to the incident light, which causes diffuse scattering, and combined with the additional scattering from the RPE and the poor penetration of light into the choroid, the signal from the choroid is attenuated resulting in an inability to differentiate flow within these large choroidal vessels, as shown in Figure 9-3J.
The retinal vascular projection artifacts (or tailing artifacts) shown in Figure 9-3H are clearly a drawback of all OCTA techniques because these artifacts can obscure the signals from neovascular blood vessels located in the outer retinal space, which are found typically in age-related macular degeneration (AMD). To mitigate this problem, Zhang et al21 recently proposed a practical and automatic projection artifact removal algorithm that has been demonstrated to successfully remove these projection artifacts efficiently, which is important for OCTA imaging of macular neovascularization (MNV).
CLINICAL PERFORMANCE OF OPTICAL MICROANGIOGRAPHY IMAGING
For almost half a century, dye-based angiography has been the mainstay in ophthalmic imaging for the diagnosis and monitoring of ocular diseases with vascular involvement. However, the need for an intravenous injection of contrasting dyes, the relatively long procedure time (> 10 minutes), and the risks associated with these dyes make them less than ideal for routine and repeated use in patients. In contrast, OCTA provides an ability to visualize functional ocular vessels by detecting the differences between cross-sectional B-scans without a need for contrasting dyes, which permits repeated longitudinal monitoring of disease progression and therapeutic treatment outcomes. Moreover, OCTA is more time efficient. It takes only a few seconds for OCTA to complete one volumetric scan. Furthermore, OCTA provides the visualization of vasculatures in 3 dimensions with near-microscopic resolution, albeit with a limited field of view. Though wide-field FA and ICGA are possible to achieve, the result is still two-dimensional; therefore they cannot provide depth information of vascular networks without 3D viewing, and even then, the resolution is poor. As a depth-resolved imaging technique, OCTA allows for visualizing vascular networks at various depths, making it possible to investigate specific retinal and choroidal layers where disease originates and propagates. In addition, the high axial and transversal resolution of OCT provides sufficient resolving power to detect capillary vessels, which is a real advantage over traditional angiography.
Owing to the above attributes, OCTA/OMAG has been rapidly moved into the clinic for the investigation of a number of retinal diseases such as DR (eg, Zhang et al15), glaucoma (eg, Jia et al22), MacTel2 (eg, Zhang et al18), branch retinal vein occlusion (eg, Kashani et al23), and AMD (eg, Miere et al24). Over a period of 2 years, between 2013 and 2015, hundreds of individuals have been recruited with normal and diseased eyes at Bascom Palmer Eye Institute (Miami, Florida) and the University of Washington Eye Institute (Seattle, Washington). There are 2 OCT angiography system prototypes involved in the collection of datasets from patients to generate OMAG angiograms. One is a Cirrus spectral domain OCT angiography (SD-OCTA) prototype system, operating at 840 nm central wavelength. The other is a Cirrus swept source OCT (SS-OCT) angiography prototype system, operating at 1050 nm central wavelength. Both systems are from Carl Zeiss Meditec Inc.
In the following examples, we show how OMAG images perform when generated from normal and pathological eyes. For the results presented here, the study was reviewed and approved by the respective institutional review boards at the University of Washington and the University of Miami Miller School of Medicine. Informed consent was obtained from all participants before imaging. This research followed the tenets of the Declaration of Helsinki and was conducted in compliance with the Health Insurance Portability and Accountability Act of 1996.
Advantages of the FastTrac
To achieve high-quality and high-fidelity OMAG angiograms, the motion artifacts caused by constant tissue motion should be minimized. The FastTrac technique is available on the Cirrus SD-OCTA and SS-OCTA prototypes that use an laser scanning ophthalmoscope to trace the eye motion and guide OCT scanning, which enables motion-free OMAG angiograms.19 Figures 9-4A to 9-4C depict motion artifacts caused by the microsaccades with- out using motion tracking. The motion artifacts, appearing as white horizontal lines, cause the discontinuities and distortions (the yellow rectangles) of the functional blood vessels in all the retinal layers of OMAG angiograms. However, with FastTrac, these artifacts no longer appear, resulting in motion-free angiograms with distinct and smooth vasculature networks (Figures 9-4D to 9-4F).
Figure 9-4. En face images of the retinal vasculature obtained by optical microangiography (top) without tracking and (bottom) with tracking: (A) and (B) the en face angiograms of the superficial and deep retinal layers with artifacts that include horizontal stripes and discontinuity of the vessels (boxes), (C) the color-coded en face vascular map after merging (A) with (B). (D) to (F) En face angiograms with the motion tracking enabled. The tracking system works well in eliminating motion artifacts. The red color in (C) and (F) represents the inner retinal layer, and the green color represents the deep retinal layer. The image size is 2.4 × 2.4 mm.19 (Reprinted with permission from Zhang Q, Huang Y, Zhang T, et al, Wide-field imaging of retinal vasculature using optical coherence tomography-based microangiography provided by motion tracking. J Biomed Opt. 2015;20 [6].)
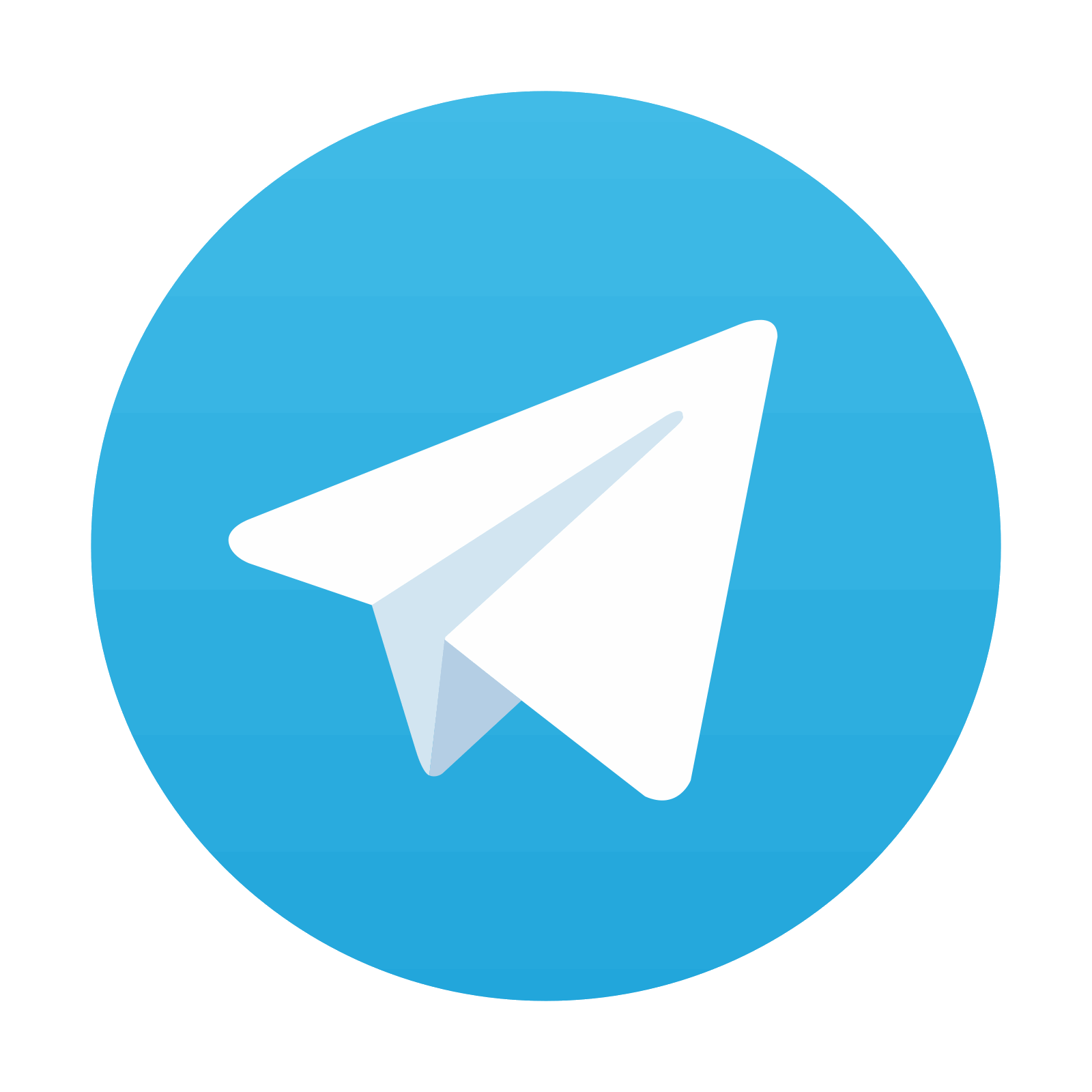
Stay updated, free articles. Join our Telegram channel
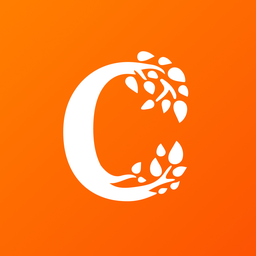
Full access? Get Clinical Tree
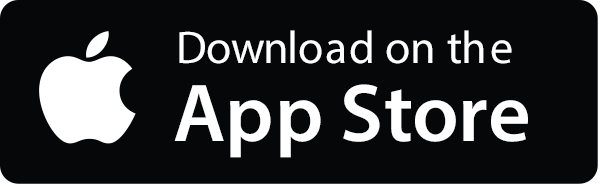
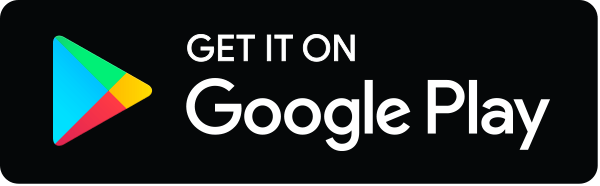