Optical Coherence Tomography Angiography Algorithms
Yali Jia, PhD; Simon S. Gao, PhD; and David Huang, MD, PhD
Optical coherence tomography (OCT)1–3 is the most commonly used imaging modality in ophthalmology. Improvements in sensitivity, acquisition speed, and resolution4–7 have enabled volumetric imaging of the anterior segment, retina, and optic nerve head with micrometer-scale depth resolution. Although conventional structural OCT aids the clinician in visualizing the anatomic changes that affect vision, it provides no direct information regarding retinal or choroidal vasculatures. As a result, structural OCT cannot identify vascular changes such as capillary dropout or pathologic new vessel growth in age-related macular degeneration and diabetic retinopathy that can lead to vision loss.
To visualize vascular changes, the most commonly used angiographic techniques in clinical practice are fluorescein angiography (FA) or indocyanine green angiography. FA is typically used to visualize retinal vasculature, while indocyanine green angiography is used for choroidal vasculature. While extremely useful, they require intravenous dye injection, which is time consuming and can have side effects.8,9 In addition, dye leakage or staining may confuse the boundaries of capillary dropout or neovascularization. Finally, these techniques provide little depth information because of the two-dimensional nature of the acquired images.
In order to develop a no-injection, dye-free method for visualizing ocular vasculature, functional extensions of OCT have been explored. These techniques aim to contrast vasculature from static tissue by assessing the change in the OCT signal caused by flowing red blood cells in blood vessels. Although the specific methodology for OCT angiography (OCTA) has changed over the years, the concept remains the same. They can be broadly classified as techniques that rely on Doppler shift or speckle variance/decorrelation. This chapter provides a historical overview of OCTA techniques for en face blood vessel and microvasculature visualization. Because Doppler OCT is now mainly used for quantifying blood flow in larger vessels and not angiography, it is only briefly mentioned. For more information on Doppler OCT, please refer to a 2014 comprehensive review.10
LABEL-FREE ANGIOGRAPHY
For more than half a century, scientists, engineers, and clinicians have collaborated to devise technologies to visualize and quantify changes in the retinal and choroidal vascular networks that supply the eye. Techniques such as color Doppler imaging, laser Doppler velocimetry, laser speckle assessment, and blue field entopic technique have provided valuable insights into retinal physiology, but have not seen wide clinical use.11 The limitations of these approaches include difficulty of use, poor reproducibility, large population variation in blood flow parameters, or limited availability of complex instrumentation. Because OCT systems are widely used in ophthalmology, its application to blood flow visualization and measurement could make clinical use more practical.
Since the early days of time-domain OCT, Doppler OCT has been explored as a tool for blood flow imaging. Doppler OCT uses the blood flow-induced phase difference between adjacent A-scans to calculate the Doppler frequency shift as the contrast between vessels and static tissue.12,13 Although Doppler OCT could measure and quantify blood flow velocity in larger vessels (Figure 2-1),14 it is not well suited for angiography of retinal and choroidal microvasculature, where vessels are nearly perpendicular to the OCT beam. Alternatively, special multibeam OCT systems can be used to introduce known Doppler angles, but such systems are complex and difficult to use.
Figure 2-1. The first demonstration of blood flow imaging in the living human eye with OCT. B-scan image of central vessels superior to the optic nerve head with Doppler signal overlaid in false color. (Reprinted with permission from Yazdanfar S, Rollins AM, Izatt JA. Imaging and velocimetry of the human retinal circulation with color Doppler optical coherence tomography. Opt Lett. 2000;25(19):1448-1450.)
Due to the slow speed of time-domain systems and the challenge posed by eye motion, volumetric angiography was not feasible until development of the 2 Fourier-domain OCT implementations: spectral domain (SD) and swept source (SS).4,15–17 With a roughly 100-fold improvement in acquisition speed, Makita et al in 2006 used an 18.7 kHz SD-OCT system to perform volumetric angiography and visualization of retinal and choroidal vasculature.18 As noted by Makita et al18 and others,19,20 the standard deviation/variance21 or power22,23 of the Doppler signal provided better results than the Doppler shift. An alternative approach called optical microangiography (OMAG) incorporated the amplitude of the OCT signal in addition to phase. An and Wang showed that OMAG (Figure 2-2) was better able to identify the microvasculature than previous methods using only phase information.24
With the continued increase in OCT system speeds, methods for OCTA shifted from comparing between adjacent A-scans to between sequential cross-sectional B-scans. The increased time separation ensured that slower flow in the microvasculature would be detected. In 2009, Fingler et al used a 25 kHz SD-OCT system and a phase-variance approach to show microvasculature (Figure 2-3) that was analogous to FA in human eyes.19 In 2011, Kim et al used a 125 kHz SD-OCT system to image with a larger field of view.20 They used montaging/stitching of 10 volumes to generate an OCT angiogram with coverage comparable to FA.
While phase-based approaches have been successful, they required precise removal of background phase noise due to bulk tissue motion or from system instabilities. Within an OCT system, phase noise can arise from scanning mirrors. In an SS-OCT system, the cycle-to-cycle variability in the spectral shift and signal acquisition produces additional noise.25,26 To compensate for bulk motion, an amplitude-weighted histogram approach could be used.27 A histogram-based Doppler shift compensation method22,28 was another option. To improve system phase stability, software or hardware modifications could be made.29,30 In an effort to circumvent the problem of phase noise compensation, OCTA based on the amplitude or intensity of the OCT signal was explored.
OCTA based on amplitude or intensity was initially described in 2005, when Barton and Stromski adapted laser speckle analysis for time-domain OCT.31 Speckle arises as a property of the interferometric nature of OCT, and speckle statistics contain information regarding the motion of scatterers.32,33 Specifically, the speckle pattern stays relatively constant over time for static objects while the pattern changes for objects in motion. Mariampillai et al extended the technique and presented speckle variance detection of microvasculature in a dorsal skinfold model using an SS-OCT system in 2008.34 In their work, speckle variance was calculated as the variance of the OCT reflectance amplitude over 3 repeated B-scans at the same location. In optimizing the method, Mariampillai et al35 noted in 2010 that the B-scan rates for repeat scans needed to be fast enough that bulk motion between B-scans was less than the OCT beam waist radius. Although “speckle variance” has been historically associated with amplitude-based OCTA, fundamentally both amplitude and phase-based flow detection are based on variation in the speckle pattern, and therefore provide largely equivalent information.36 In addition to speckle variance, another intensity-based OCTA approach was termed correlation mapping.37 In correlation mapping OCTA, cross-correlation of a grid on adjacent B-scans was performed to identify vasculature (weak correlation) vs static tissue (strong correlation).
Figure 2-2. The first demonstration of optical microangiography in the living human eye. (A) Projection image from the whole scanned volume with retinal blood vessels in green and choroidal vessels in red. (B) X-Y projection image of the blood vessels within the retina only. (C) X-Y projection image of the blood vessels within the choroid only. Scale bar = 500 μm.24 (Reprinted with permission from An L, Wang RK. In vivo volumetric imaging of vascular perfusion within human retina and choroids with optical microangiography. Opt Express. 2008;16(15):11438-11452.)
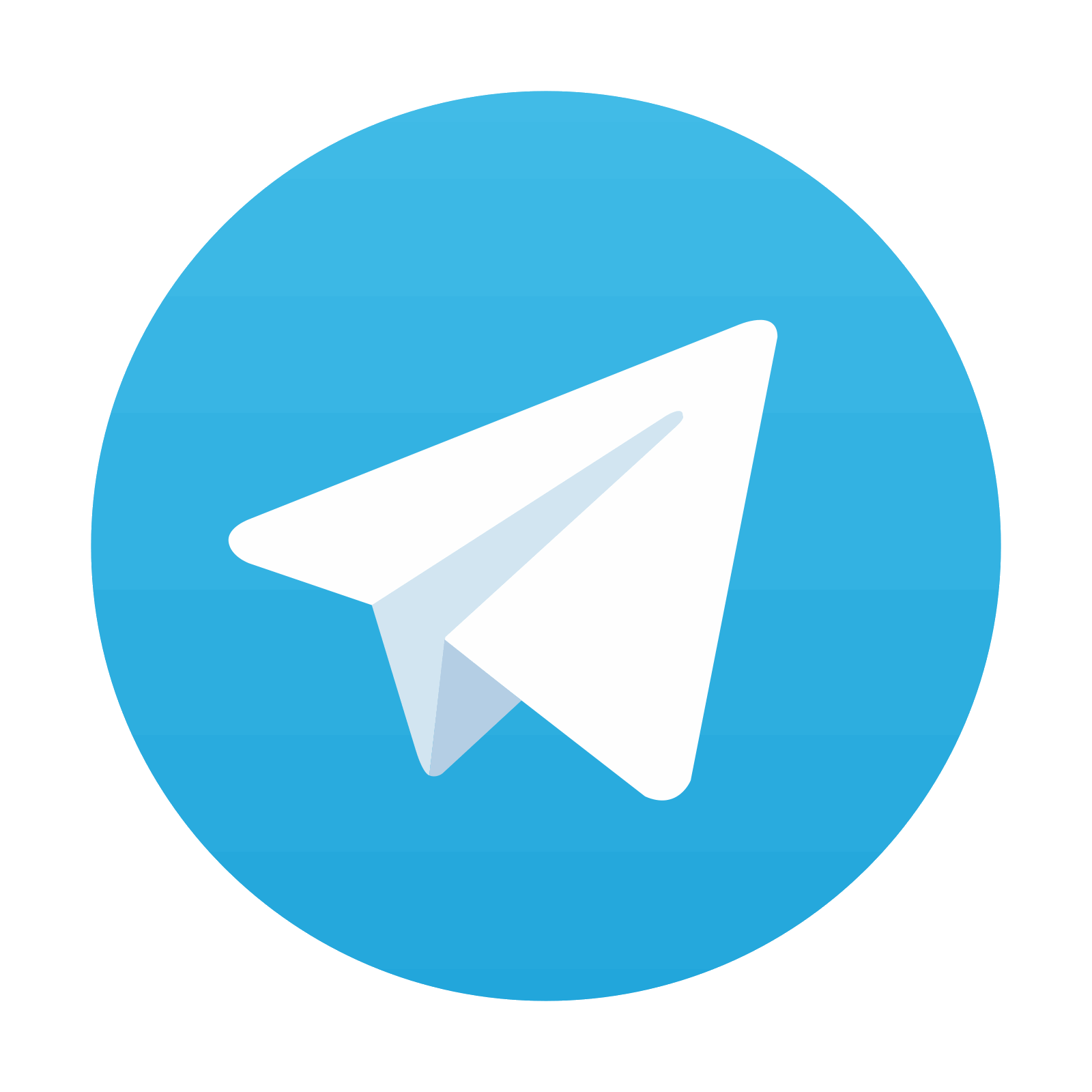
Stay updated, free articles. Join our Telegram channel
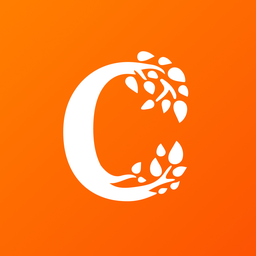
Full access? Get Clinical Tree
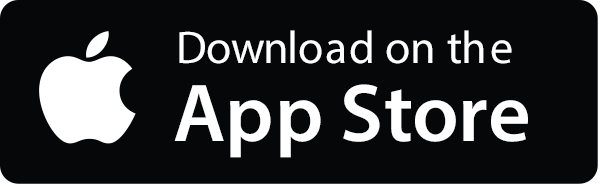
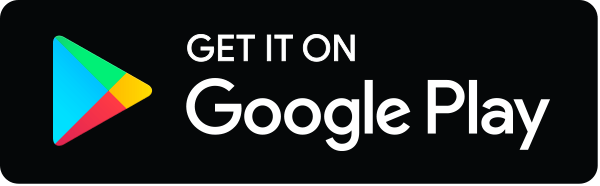