Fig. 12.1
Coronal CT image (soft tissue window) demonstrating bony anatomy and extraocular muscles of the orbit: o optic nerve, i intraconal space, e extraconal space, circle border between intraconal and extraconal spaces formed by connecting the extraocular muscles. Source Fig. 1 from van der Pol C, Chakraborty S, Nguyen T, Torres C, Glikstein R, Gao J. Imaging Anatomy and Pathology of Extraocular Muscles in Adults. Canadian Association of Radiologists Journal [serial online]. November 2014;65(4):366–371 6p. Reprinted with permission from Elsevier
As evidenced in Fig. 12.1, the orbital and paraorbital bony anatomy is easily visualized in CT imaging and can help determine if bony pathology such as fractures are present. Although MRI has significant advantages to CT as described below, CT may be the only option in cases with ferromagnetic metallic foreign bodies. The low cost of CT and ability for rapid acquisition of images make it preferable in the immediate evaluation of trauma, as its resolution still allows for adequate visualization of key structures such as the ophthalmic artery and ocular motor nerves [3].
MR Imaging of the Orbit
MRI provides the advantage over CT of improved visualization of the soft tissues without obscuration by surrounding bony structures, and additionally avoids exposing the patient to ionizing radiation. Weighting of the MRI changes how structures appear [brighter (hyperintense) or darker (hypointense)] based on time for longitudinal and horizontal relaxation of the magnetic moments. In T1 weighted images, tissues with shorter times for longitudinal relaxation will appear brighter, while those with longer times for longitudinal relaxation will appear darker. In T2 weighted images, the same concept applies but for horizontal relaxation times. Table 12.1 elaborates on which particular tissues appear bright or dark on T1 versus T2 imaging.
Table 12.1
Appearance of various tissue types in unenhanced T1 and T2 weighted MRI images
Tissue | T1 | T2 |
---|---|---|
Air | Very dark | Very dark |
Blood, acute | Dark to intermediate | Dark |
Blood, chronic | Dark rim with variable center | Dark rim with variable center |
Blood, hyperacute | Intermediate | Intermediate |
Blood, subacute | Bright rim | Bright |
Bone, cortical | Very dark | Very dark |
Bone, marrow | Bright | Intermediate |
Cortical gray matter | Dark | Bright |
Cerebrospinal fluid | Very dark | Very bright |
Fat | Very bright | Intermediate to dark |
Muscle | Dark | Dark |
Optic nerve | Dark to intermediate | Intermediate |
Proteinaceous fluid | Intermediate to bright | Intermediate |
Sclera | Dark to intermediate | Intermediate |
Vitreous | Dark | Bright |
Water | Very dark | Very bright |
White matter | Bright | Dark |
The preferred imaging in ocular trauma is currently high-resolution T1 MRI of the brain and orbits with contrast and fat suppression sequences [4]. The fat suppression prevents intraconal and extraconal fat from obscuring other enhancing lesions with its own bright signal and can be performed by using chemical shift imaging or short T1 inversion recovery sequences (STIR) [5]. The benefit of fat suppression is illustrated in Fig. 12.2 for normal anatomy.


Fig. 12.2
The effects of fat suppression in imaging of normal orbital anatomy demonstrated by axial T1 weighted MRI (a, b) and axial T1-weighted MRI with fat suppression (c, d). a Hyperintense fat around normal structures produces an artifact (arrow) that causes the optic nerve to appear enlarged (arrowheads). b Hyperintense fat obscures visualization of the lacrimal gland (between arrows). c Fat suppression better reveals the optic nerve (arrowheads) against low-intensity CSF. d Fat suppression also increases the contrast between the lacrimal gland (between arrows) and surrounding structures, and allows for visualization of the superior ophthalmic vein (arrowhead), which is now hyperintense compared to the suppressed fat. From J Simon, J Szumowski, S Totterman, D Kido, S Ekholm, A Wicks, and D Plewes. Fat suppression MR imaging of the orbit. AMNR 1988 9:961–8. Reprinted with permission from American Journal of Neuroradiology
As seen in Fig. 12.2, axial images can be used to evaluate many of the structures of the orbit, such as the optic nerve and superior ophthalmic vein. The level of the axial slice can demonstrate some structures together in one image, such as the lateral and medial rectus muscles if the slice is taken at the level of the optic nerve. Axial images can also be used to identify important structures before and during their passage through the orbital apex, as in Fig. 12.3, where inflammatory changes at the left orbital apex can be visualized.


Fig. 12.3
Axial T1 image with gadolinium and fat saturation of the orbits showing enhancement of the soft tissue structures in the left orbital apex. This patient presented with painful ophthalmoplegia and proptosis. Source From Ferreira et al. [262]. Reprinted with permission from Science Direct
Mid-sagittal MR images of the orbit are able to demonstrate the globe, extraocular muscles, optic nerve, and surrounding fat and bony structure. In the sagittal image in Fig. 12.4, the superior and inferior rectus muscles as well as the inferior rectus and orbicularis oculi muscles are dark grey structures shown along their course to the globe. Sagittal images taken more lateral or medial would demonstrate more of the extraocular muscle anatomy and omit the optic nerve (not shown).


Fig. 12.4
Mid-sagittal T1 MRI, lateral view of the orbit. Orbital contents: 1 orbicularis oculi muscle, 2 globe, 3 inferior oblique muscle, 4 retrobulbar (extraconal) fat, 5 inferior rectus muscle, 7 superior rectus muscle, S superior ophthalmic vein, M maxillary sinus, CN II optic nerve. From Moore K, Dalley A, Agur A. Clinically Oriented Anatomy [e-book]. Philadelphia: Wolters Kluwer Health/Lippincott Williams and Wilkins, 2010; 2010. Available from: SUNY Downstate Brooklyn—Catalog, Ipswich, MA. Reprinted with permission from LWW
T2-weighted gradient refocused-echo (GRE) imaging appeared to improve the evaluation of diffuse axonal injury (DAI) in TBI [6]. In the comparison study between T2 and T2-GRE imaging, more focal traumatic hemorrhages were seen on the GRE imaging as depicted in Fig. 12.5.


Fig. 12.5
Left T2-weighted image of a 42M in a MVA suffering traumatic microbleeds evident in the posterior corpus callosum; Right T2-GRE image revealing additional traumatic microbleeds in the left side of the splenium and the gray–white matter junction of the frontal lobes. From Scheid et al. [6]. Reprinted with permission from American Journal of Neuroradiology
Optic Nerve Trauma
Traumatic Optic Neuropathy (Basic Definitions)
Cranial nerve II, the optic nerve, transmits afferent visual inputs from the retina that eventually reach higher cortical processing centers in the occipital lobe. In traumatic optic neuropathy (TON), complete or partial impairment of visual function follows orbital or head trauma due to damage of optic nerve fibers [7, 8].
Direct or indirect forces may damage the optic nerve. Blunt trauma to the head or orbit is the more common cause of TON, termed indirect TON [9]. The force of closed head trauma is transmitted through the surrounding soft tissue and bony optic canal to the optic nerve, disrupting optic nerve fibers [10, 11]. In direct TON, penetrating injury causes direct stress to the optic nerve with resultant anatomical disruption [9, 10, 12]. Direct TON is less common than indirect TON and has a worse prognosis for long-term visual potential [7].
Optic Nerve Anatomy
There are four anatomical divisions of the optic nerve: intraocular (1 mm), intraorbital (24–28 mm), intracanalicular (9 mm), and intracranial (16 mm) (see Fig. 12.6) [11].


Fig. 12.6
Optic nerve segments. From Yanoff M, Duker JS (eds) Ophthalmology, 2nd edn. St Louis, Mosby, 2004. Reprinted with permission from Elsevier
Intraocular
The intraocular ON is comprised of the optic disc, prelaminar area (anterior to lamina cribrosa), and laminar area. Beginning at the optic disc, 1.2 million retinal ganglion cell (RGC) axons exit the globe through the scleral canal to form the optic nerve. The RGC axons are physically supported by the lamina cribrosa and metabolically supported by interweaving astrocytes [9]. The lamina cribrosa is a system of 10 connective tissue plates integrated with the sclera, and axon bundles are transmitted through openings in the lamina cribrosa.
Intraorbital
Posterior to the lamina cribrosa, the optic nerve fibers are myelinated by oligodendrocytes and surrounded by a meningeal sheath (pia mater, arachnoid mater, and dura mater). At this point, the optic nerve is within the muscle cone of the eye. The intraorbital part of the optic nerve is longer than the distance between the posterior globe and optic canal. This length difference allows for optic nerve laxity in the orbit and facilitates unrestricted globe rotation. Before entering the optic canal, the optic nerve passes through the annulus of Zinn.
Intracanalicular
The optic canal travels through the lesser wing of the sphenoid bone. In the optic canal, the dural sheath surrounding the optic nerve fuses with the periosteum and immobilizes the nerve.
Intracranial
At the transition from intracanalicular to intracranial segments, the optic nerve passes under a dural fold called the falciform ligament. The final intracranial portion of the optic nerve does not have a dural sheath. The optic nerve terminates at the optic chiasm where the neural fibers continue posteriorly as the optic tracts.
Vascular Supply
(see Fig. 12.7). The short posterior ciliary arteries, branches from the ophthalmic artery, provide vascular supply to the optic nerve head. The short posterior ciliary artery distribution has few anastomoses, so the optic nerve head is susceptible to ischemia in cases of poor perfusion [13]. The primary blood supply along the length of the optic nerve is from pial branches of the surrounding meninges. The branches are supplied by the posterior ciliary arteries, which are small branches from the ophthalmic artery. Although the central retinal artery and vein travel within the anterior 10–12 mm of the optic nerve, only a small portion of optic nerve vascular supply is from the central retinal artery (see Table 12.2 and Fig. 12.7).


Fig. 12.7
From Balcer LJ and Prasad S, “Abnormalities of the Optic Nerve and Retina”. Reprinted with permission from Springer Publishing Company
Table 12.2
Regional differences in vascular supply to the optic nerve
Segment | Blood supply |
---|---|
Intraocular Optic disc Prelaminar Laminar | Retinal arterioles Branches of posterior ciliary arteries |
Intraorbital | Intraneural branches of central retinal artery; pial branches from CRA and choroid |
Intracanalicular | Ophthalmic artery |
Intracranial | Branches of internal carotid and ophthalmic arteries |
Optic Nerve Anatomy—Clinical Application to Trauma
The clinical picture of TON varies based on the anatomical site of injury. In posterior optic nerve injury, the most common injury, the optic nerve is damaged posterior to the entry point of the central retinal vessels [12]. In anterior optic nerve injury, a less common presentation, damage is anterior to where the central retinal artery enters the optic nerve. In this injury, visual loss is often associated with abnormalities in the retinal circulation [8].
The most common site of optic nerve injury is intracanalicular. In a study of 42 patients with TON, 71.4% of injuries occurred at this location [11, 14]. The dural sheath of the optic nerve is adherent to the periosteum in the optic canal, and such areas of dural sheath attachment are highly susceptible to trauma [14, 15]. Coup and contrecoup forces in blunt head trauma cause the greatest degree of injury at transition sites between mobile and fixed segments of the nerve [16]. Traumatic stress disrupts the pial vessels within the optic canal, and neuropathy may result from decreased vascular supply to the optic nerve [16]. In addition, the majority of blunt forces from frontal head injury are transmitted to the optic foramen [14, 17]. With the direct trajectory of force and the closed, inflexible space of the optic canal, there is higher risk of compression, shearing, contusion, and stretching injuries to the intracanalicular optic nerve [17].
The optic nerve is also susceptible to traumatic forces in the intracranial segment where the optic nerve passes under the falciform dural fold [8]. The falciform dural fold may impinge on the optic nerve and produce shearing forces during blunt trauma [18]. Still, the intracranial optic nerve is relatively shielded from injury by the surrounding soft tissue and bone. Shearing forces are first absorbed by the intracanalicular optic nerve and often do not reach the intracranial segment [17].
Comparatively, injury of the intraocular optic nerve segment is rare. The intraorbital segment of the optic nerve is usually spared from injury due to its laxity within the orbit and shielding by the surrounding fat and extraocular muscles [17].
TON Pathophysiology
There is an evolving understanding of the pathophysiology of TON, which is likely multifactorial [19, 20]. One perception of indirect TON describes primary and secondary injuries. With primary injury, the immediate outcome of trauma is contusion necrosis [8]. There is irreversible shearing of RGC axons and subsequent RGC degeneration [8, 12, 21]. Secondary injury is caused by optic nerve edema within the rigid optic canal, which produces a compartment syndrome [9]. Edema may be driven by the initial trauma or the resultant ischemia. The optic nerve is highly susceptible to edema from ischemia, inflammation, or compressive forces because its axonal transport system has a high-energy requirement and is easily disrupted. The compartment syndrome exacerbates ischemia by further limiting blood supply to the RGCs, and many RGCs undergo apoptosis [12]. Because primary injury is irreversible, TON treatments focus on limiting secondary injury. Evolving protocols include neuroprotection strategies and medical or surgical decompression to alleviate the edema [21–23].
Epidemiology and Mechanism
A large 2009 survey of patients in the UK estimates the minimum incidence of TON as 1 per one million people per year [24]. TON occurs secondary to head injuries (see Table 12.3). In prospective studies, the incidence of TON following head trauma varies between 0.5 and 5% of closed head injuries [8, 19, 20]. In adult patients, the predominant mechanisms of TON include falls, assault, and motor vehicle accidents (MVAs; see Table 12.3). In pediatric populations, mechanisms for TON are similar and primarily include falls from a height, MVAs, and sports injuries [25, 26]. TON is more frequently diagnosed in men and younger patients, averaging around 30 years old [7, 24, 27–29]
Table 12.3
Reports on common mechanisms of TON
Author/References | Cases | MVA/bicycle accident | Fall | Assault | Other |
---|---|---|---|---|---|
Pirouzmand [27] | 50 | 32 (64%) | 12 (24%) | 5 (10%) | 1 (2%) |
Urolagin [32] | 8 | 7 (88%) | – | – | 1 (12%) |
Roccia [37] | 14 | 5 (36%) | 4 (28%) | 1 | 4 (28%) |
Lee [24] | 121 | 26 (22%) | 31 (26%) | 26 (20%) | 38 (31%) |
Lubben [40] | 65 | 30 (46%) | – | 7 (11%) | 33 (51%) |
Kountakis [41] | 34 | 19 (54%) | 9 (26%) | 5 (15%) | – |
Levin (IONTS) [28] | 133 | 58 (46%) | 27 (21%) | 18 (14%) | 24 (19%) |
Joseph [42] | 14 | 5 (35%) | 4 (29%) | 3 (21.4%) | 2 (14%) |
Seiff [43] | 36 | 15 (42%) | 13 (36%) | 4 (11%) | 4 (11%) |
Spoor [44] | 21 | 10 (48%) | 3 (14%) | 8 (38%) | 0 (0%) |
Lessell [29] | 33 | 22 (67%) | 8 (24%) | 2 (6%) | 1 (3%) |
Millesi [45] | 29 | 18 (62%) | 6 (21%) | 5 (17%) | – |
Nau et al. [39] | 18 | 9 (50%) | 5 (28%) | 1 (6%) | – |
Anderson [14] | 7 | 4 (57%) | 2 (12%) | – | – |
Matsuzaki [46] | 33 | 20 (60%) | 7 (21%) | 4 (12%) | 2 (6%) |
Bodian [47] | 6 | 4 (67%) | 2 (33%) | – | – |
Total | 622 | 284 (46%) | 133 (21%) | 89 (14%) | 110 (18%) |
In patients with mid-facial fractures, 2.5% develop TON [30]. The likelihood of TON increases with more severe head trauma. There is 6–10% rate for patients with zygomaticomaxillary complex fractures [19, 31, 32], and patients with nasoethmoid complex fracture have 1.6 times greater chance of developing TON [27].
Less common causes of TON in adults are secondary to recreation-associated trauma during sports or iatrogenic [24]. Iatrogenic TON has been identified secondary to orbital surgery [33], osteotomies [34, 35], facial fracture repair [36, 37], and endoscopic sinus surgery [32, 38].
Other serious injuries often coexist, including orbital wall fractures, closed globe injuries (both anterior and posterior segment), ocular adnexal injuries to eyelid, extraocular muscles, orbital foreign body, skull fractures, and intracranial bleeding [24, 29]. However, it is difficult to predict the likelihood of TON solely based on the severity of the trauma [39].
Diagnostic Work-Up
History
The diagnosis of TON is primarily clinical [9, 10]. There are often delays to diagnosis if the patient presents with impaired consciousness or unstable condition secondary to multisystem trauma [10, 19, 48]. The history frequently reveals a mechanism of head trauma, as stated above, followed by subjective decrease in visual acuity. Detailed questions should be asked about the mechanism of trauma, including the potential for ocular foreign bodies with penetrating injury. Determine the time course for visual change and time when the injury occurred [11]. Also investigate the patient’s past ocular history, including any previous trauma or surgery to the involved eye.
Physical Examination
If there is suspicion for TON, assess pupillary reactivity, visual acuity (VA), visual fields, and color vision, perform a funduscopic examination on all patients. Examination may be limited due to lid ecchymosis, edema, or other extensive facial trauma [49].
Pupillary Examination
The most reliable early sign of optic nerve dysfunction is the presence of a relative afferent pupillary defect (RAPD) [20]. A RAPD will be present with the exception of bilateral and symmetric TON [10, 11]. This can be examined regardless of a patient’s level of consciousness, so it is an invaluable measure for the diagnosis of TON in unconscious patients [39].
Visual Acuity Testing
Patients with TON often have an acute decrease in VA following trauma. Occasionally, decrease in VA is delayed over weeks secondary to inflammation and edema or development of an optic nerve sheath hematoma [8, 20]. Visual acuity is often 20/400 or less in the affected eye [10]. In studies, 40–60% of patients initially present with light perception or worse [24, 32, 44].
Visual Field Testing
Variable visual field defects are observed following TON. Commonly observed visual field defects following TBI are scattered visual field loss (58%) and homonymous visual field defects (22%) measured by confrontation method [50, 51]. If there is partial optic nerve avulsion, visual field loss will correspond to the location of injury [15].
Color Vision Testing
Color vision is often decreased in TON. In one report, 76% of patients with TON visualized 12/17 or fewer Ishihara color plates [24].
Fundus Examination
Findings of a RAPD and a normal fundus examination increase the likelihood of TON. In posterior optic nerve injury, the fundus appears normal during the early clinical stage [12, 24]. With an anterior optic nerve injury, there may be optic disc swelling, dilated and tortuous retinal veins, or retinal hemorrhages [11, 15, 24]. Optic atrophy develops over the following weeks, and becomes evident 4–6 weeks after injury [9, 11, 19]. Therefore, it is useful to monitor patients with repeated dilated fundus examinations following closed head trauma. Of note, the fundus examination may initially be difficult to complete if there is coexisting intraocular hemorrhage (IOH), thus a repeat fundus examination should be performed at a later date in these instances.
Additional Physical Examination
Assess ocular adnexa for other effects of trauma, including crepitus or step-off from orbital fractures [10]. Evaluate for oculomotor dysfunction, including ability to perform extraocular movements, strabismus, and accommodative and vergence defects [52]. Identify proptosis, which may be associated with intraorbital hemorrhage and can be difficult to diagnose if there is also periorbital swelling. Complete a full ophthalmic examination to identify additional ocular consequences of head trauma, such as corneal abrasion, traumatic cataract, and vitreous prolapse [53].
Imaging
Imaging has uncertain clinical value. There is no consistent correlation between the finding of an optic canal fracture, the severity of visual loss, and the prognosis for visual recovery [17].
Computerized Tomography (CT)
CT is the best imaging tool to identify optic canal fracture, intracranial mass lesion, such as a hematoma, subluxated lenses, or other specific pathology compromising the optic nerve [11, 19]. There is no clear protocol for imaging studies in the diagnosis of TON. Some clinicians use CT scan for all cases, while others reserve imaging based on clinical severity or when contemplating the need for therapeutic interventions [20]. For example, CT imaging is part of surgical planning for optic nerve decompression [10, 49].
Magnetic Resonance Imaging (MRI)
MRI is an alternate imaging modality to assess intracranial mass lesions. It is used for patients with a clear history of trauma and no likelihood of metallic foreign bodies. However, MRI may be difficult to perform in critically injured patients [19].
Ultrasound
B-scan ultrasonography is an inexpensive imaging modality that can be used in closed orbit injury [11]. It has demonstrated utility for early diagnosis of traumatic optic nerve avulsion. Optic nerve avulsion is often associated with concomitant vitreous hemorrhage that obstructs visualization of the fundus, and B-scan allows visualization despite intraocular bleeding [54].
Visual Evoked Potentials (VEP)
Flash visual evoked potential (FVEP) is a useful form of VEP because it can be performed in patients despite level of consciousness, lid hematomas, or refraction abnormalities [39]. VEP should be measured in both eyes, with the uninjured eye as a control [11]. The presence and amplitude of VEP predicts visual outcome in TON [55]. Deformed evoked potentials correlate with morphological changes of the optic nerve and measurements of reduced pupillary reactivity [39].
Fluorescein Angiography (FA)
Prognosis
In patients with indirect TON, there is a correlation between initial and final visual acuity. Patients who are NLP on presentation have little or no visual acuity improvement regardless of medical, surgical, or conservative management [7, 43, 58]. If there is no visual recovery after 48 h, the prognosis for final visual improvement is poor [20, 59]. Furthermore, younger patients have greater likelihood of visual recovery [60].
Measurements of the RAPD with neutral density filters indicate that patients with an initial RAPD of 2.1 log units or less can have visual acuity improve to 20/30 or better, while patients with an initial RAPD of 2.1 log units or greater have little visual improvement [17, 61].
Loss of consciousness following trauma is associated with a poor visual prognosis [20]. This finding may be related to a higher impact injury. Similarly, the presence of an orbital fracture is associated with smaller improvements from initial to final visual acuity due to a greater force of traumatic impact and greater severity of optic nerve trauma [7, 62]. Patients with anterior orbital fractures have more visual acuity recovery than patients with posterior orbital fractures [62]. Patients with optic canal fractures have varied success with visual recovery [19, 20].
VEP can also predict patient prognosis for visual function. FVEP amplitudes that are 50% of normal greater predict a good visual outcome of 20/30 or better (compared to the uninjured eye) [20]. Initial absence of visual evoked responses is associated with minimal, if any, visual recovery and final visual acuity of 20/300 or less [19, 55].
Examples of Direct TON
Direct trauma to the optic nerve may result from fractures of the optic canal, injury, or compression by bony fragments (Fig. 12.8), penetrating foreign bodies, or mass effect such as an expanding hematoma [63]. The causes of direct optic nerve injury can be distinguished with physical examination and imaging techniques. Generally, the diagnosis of direct TON is best detected radiographically with CT [63].


Fig. 12.8
Case study. This patient is status post gunshot wound to the face. On presentation, VA NLP OU. Bone fragments identified in the right orbit (arrows) causing direct trauma to the anterior medial intraorbital segment of the optic nerve. Severely traumatized left ruptured globe required primary enucleation with implant
Traumatic optic nerve avulsion
Optic nerve avulsion is a rarely reported form of anterior optic nerve injury [54]. Due to the difficulty with diagnosis, it is likely that the incidence of traumatic optic nerve avulsion is underreported. During trauma, the intraorbital optic nerve is forcibly separated from the globe at the lamina cribrosa, while the optic nerve sheath and adjacent sclera remain intact [54, 56, 65]. Avulsion may be partial or complete. In partial avulsion, there is segmental detachment of the optic nerve. In complete avulsion, there is full separation of the optic nerve from the retina, choroid, and vitreous and retraction of the lamina cribrosa from the scleral rim [56].
Mechanism
Optic nerve avulsion may follow a wide variety of injuries, including severe facial trauma, blunt injury of the orbit, and globe concussion [54, 65]. A frequent mechanism of optic nerve avulsion is direct trauma to the globe in which a small foreign body enters the space between the orbital rim and the globe to displace the eye [56, 66, 67]. For example, this can occur from a strike in the eye by a finger during contact sports such as basketball or boxing [66, 68].
Pathophysiology
Optic nerve fibers at the disc are susceptible to shearing forces as they pass through the lamina cribrosa because there is little supportive connective tissue in this location [69]. Suggested reasons for optic nerve tears at the lamina cribrosa refer to the traumatic forced rotation of the globe and resultant shearing forces, sudden increase in intraocular pressure driving the nerve posteriorly out of the scleral canal, or displacement of the globe concurrent with retropulsion of the nerve [9, 57, 69].
Clinical Presentation
Acute, severe vision loss immediately follows orbital trauma [9]. Patients are often NLP on presentation with a RAPD. Because the anterior ciliary vasculature is maintained, the globe will have a normal appearance.
Diagnosis
Fundus examination: The most specific means for diagnosis is fundoscopy (see Fig. 12.9). There is often a ring of hemorrhage encircling the visibly disrupted optic nerve head [8, 9, 15]. In the case of a partial avulsion, there are segmental depressions or excavation of the optic nerve head [56]. In complete avulsions, there is absence of the optic nerve head with a remaining “cavity” in its place [9, 54, 56]. There are often limitations to the fundus examination. The clinical diagnosis may be delayed in its earlier stages by extensive vitreous hemorrhage, hyphema, or retinal hemorrhage that obstruct a clear view of the fundus [56, 57, 68].


Fig. 12.9
Fundus photography demonstrating traumatic optic nerve avulsion. From Corrales and Curreri [66]. Reprinted with permission from Elsevier
Ultrasonography: Positive findings with B-scan include visualizing that the optic nerve does not reach the optic disc, hyperlucency anterior to optic nerve, and posterior ocular wall defects in the region of the optic nerve head (see Fig. 12.10) [54, 65]. At 4–6 weeks after injury, optic nerve head defects and ingrowth of glial scar tissue have been identified [70]. B-scan is especially useful in cases of vitreous hemorrhage or other obscuration of fundoscopy. However, B-scan has demonstrated variable success in the diagnosis of optic nerve avulsion [67].


Fig. 12.10
B-scans demonstrating hypolucencies posterior to optic nerve head (arrows). From Sawhney et al. [54]. Reproduced with permission from Nature Publishing Group
Fluorescein Angiography: The retinal vasculature may be intact or have varying degrees of interruption following either partial or complete optic nerve avulsion. There may be a spectrum of normal filling, delayed filling, partial filling, or complete absence of retinal circulation [56].
Treatment
Optic Nerve Transection
Mechanism Optic nerve transection follows direct penetrating injury to the optic nerve. Transection may be complete or partial.
In cases of mid-facial blunt trauma, compressive forces directed on the superior orbital rim are transmitted to the orbital roof and optic canal [8, 14]. The forces can fracture the optic canal or orbital bones. Bony fragments are at risk of transecting the optic nerve (see Fig. 12.11) [9, 57]. Optic nerve transection may also follow injury from a penetrating foreign body.
Fig. 12.11
Optic nerve transection (arrow)
In a review of the mechanisms of penetrating ocular trauma, optic nerve injury was most frequently caused by intentional self-inflicted injury. Common mechanisms of intentional penetrating eye injury included projectiles and blunt trauma (38%), projectiles (31%), and sharp objects (25%). Overall, penetrating eye injury is most frequently associated with projectiles (47%) [71].
Clinical Presentation
Patients will have acute visual acuity loss and a RAPD. Early funduscopic examination is often normal, and optic nerve atrophy may be observed days following trauma (Fig. 12.12).


Fig. 12.12
Optic nerve transection (arrows in Fig. 12.11). Fundus photograph prior to the trauma (left) shows normal appearing optic disc. Fundus photograph several weeks after injury (right) reveals optic atrophy with peripapillary pigmentary changes
Diagnosis
Diagnosis is largely based on clinical history of trauma, RAPD and acute vision loss with NLP visual acuity. CT scan may show a foreign body or bone fragment transecting the optic nerve (Figs. 12.13, 12.14 and 12.15) and VEP amplitudes are decreased [9].




Fig. 12.13
23-year-old-male collided with an SUV while riding a motorcycle. He had a fixed, dilated left pupil. Fractures to the medial orbit, sphenoid sinus roof, and bilateral optic canal fractures involving all 4 walls of the optic canal. On left, bilateral optic canal fractures (arrows). On right, fracture involving superior orbital fissure (arrow)

Fig. 12.14
CT scan of the head at the level of the optic canal with optic canal fracture in the left lateral sphenoid wall (arrow). From Walsh and Hoyt’s Clinical Neuro-Ophthalmology, Chapter 9, pg. 436. Reproduced with permission from Robert Alan Goldberg, MD, University of California Los Angeles

Fig. 12.15
CT scan of orbit with right optic nerve transection following penetrating trauma. From Sarkies 2004. NJC. Neuro-ophthalmological aspects of head injury. From Macfarlane R and Hardy D (eds) Outcome after head neck and spinal trauma. Butterworth Heinemann: Oxford, 1977 pp 163–177. Reproduced with permission from Elsevier
Management
Hemorrhage and Hematoma
Hemorrhage with the potential to cause direct TON may involve the optic nerve sheath, the optic nerve, or the potential space between the optic nerve and optic nerve sheath. In an autopsy series following patients with closed head trauma conducted by Crompton et al., 83% of autopsies had evidence of optic nerve sheath hemorrhage and 36% had hemorrhage involving the interstitium of the optic nerve [18].
Pathophysiology
Clinical Presentations
Diagnosis
A CT scan can identify an optic nerve sheath hematoma (Fig 12.16). The affected optic nerve sheath will be expanded and hyperdense, and its increased size and density can be visualized [9, 74]. MRI is more specific than CT scan in detecting and localizing optic nerve hemorrhage [76].


Fig. 12.16
Case study. Left optic nerve sheath hemorrhage following a forceful poke to the left eye while playing basketball. On presentation, he was NLP OS, pupils were 4 mm OD and 5 mm OS with a left RAPD, and he was unable to supraduct the left eye. a Coronal CT scan of the orbit with increased size of the left optic nerve, from hematoma. b Axial CT scan of orbit shows enlarged left optic nerve and left proptosis. He underwent emergent optic nerve sheath decompression without subsequent improvement of vision
Management
Optic nerve sheath or intrasheath hemorrhage benefits from surgical drainage [15]. In optic nerve sheath hematoma, early nerve sheath fenestration to evacuate the clot is associated with greater recovery of visual acuity [9, 74, 77]. Megadose steroids have been used to treat optic nerve sheath hematoma, but their utility is unclear (See: Indirect TON Treatment section for discussion about corticosteroid use in TON) [75].
Orbital Compartment Syndrome
Orbital compartment syndrome describes an acute increase of intraorbital pressure. It may arise secondary to orbital hemorrhage or orbital emphysema. Because a fascial sheath and rigid bony walls enclose the orbital contents, small increases in intraorbital volume from blood or air can elevate the intraorbital pressure [37]. Arterial supply to the optic nerve can be compromised and lead to optic neuropathy [9, 15].
Mechanism
Orbital hemorrhage can follow facial injury or surgery. Injection of retrobulbar anesthesia is a well-documented iatrogenic cause of orbital hemorrhage, occurring in 0.44–3% of cases [15].
Orbital emphysema is a common complication of orbital fracture, occurring in 50% of cases [78]. Nose blowing, coughing, sneezing, vomiting, or straining while lifting heavy objects by a patient with a blowout fracture can force air from the sinuses into the orbital space [78, 79]. Orbital emphysema is largely a benign process. However, air may become trapped in the orbit if a small fracture creates a one-way ball-valve mechanism [9, 15]. The trapped air increases intraorbital pressure and has a compressive, mass effect on the optic nerve [78, 80].
Clinical Presentation
Diagnosis
Diagnosis is primarily clinical, in association with a history of facial trauma or recent orbital surgery. CT scan is useful to evaluate the fracture, identify presence of orbital emphysema or hemorrhage, and localize air pockets [80]. Facial radiographs may also reveal air within the orbit, but it is best confirmed with CT scan [80].
Management
The goal of management is to rapidly decrease orbital pressure. Emergency surgical decompression by lateral canthotomy or cantholysis is essential [73, 78, 80]. The maximum potential for visual recovery follows surgical decompression within 2 h of injury [81]. In traumatic orbital compartment syndrome from retrobulbar hemorrhage, successful orbital decompression is achieved with lateral canthotomy and inferior cantholysis. When performed promptly, the patient may recover full visual function [82].
Examples of Indirect TON
Treatment of Indirect TON
The treatment of indirect TON is currently debated. Before any treatment is initiated for TON, other treatable causes of visual loss should be ruled out [10, 83]. Approaches to indirect TON include conservative management, corticosteroids, optic canal decompression, and combined surgical and steroid treatment. In addition, research for neuroprotection strategies designed to repair damaged RGCs is ongoing.
Conservative Management
With conservative management for indirect TON, patients are observed for visual recovery. Interventions such as steroids or surgery are withheld [19]. Reports of spontaneous visual recovery following indirect TON are encouraging for conservative management, with a recovery rate of 30–60% [12, 20, 43, 83, 84]. The prognosis for spontaneous recovery is positively related to the patient’s presenting visual acuity [12, 20]. A prospective study is needed to more clearly define the natural course of indirect TON [8, 83].
Corticosteroids
There is mixed evidence regarding the use of corticosteroids to treat indirect TON. High-dose corticosteroids administered soon after trauma may relieve optic nerve edema within the optic canal and decrease risk of damage to RGCs [14]. However, research outcomes have shown unclear benefits with corticosteroid treatment. There is debate on the best dose and time course of treatment. In addition, the risks of long-term disability and mortality from high-dose steroid regimens must be carefully considered.
In 1990, the multicenter, randomized, double-blind controlled National Acute Spinal Cord Injury Study (NASCIS 2) examined the use of “megadose” methylprednisolone following acute spinal cord injury [85]. An experimental group of patients with acute spinal cord injury was administered a bolus methylprednisolone dose of 30 mg/kg followed by infusion at 5.4 mg/kg/h for 23 h, while a control group received a placebo. Neurologic recovery was assessed with measurements of motor function, sensation to pinprick, and sensation to touch. Patients were followed up to 6 months after injury. The researchers found that patients who received methylprednisolone within 8 h of their injury had significant neurologic recovery, and this recovery remained stable when assessed 6 months following injury. Neurologic recovery was not observed in the placebo group or patients who received methylprednisolone greater than 8 h following trauma [85].
In a subsequent multicenter, randomized, double-blind clinical trial, NASCIS 3, researchers examined the outcome of extending the duration of megadose methylprednisolone treatment. Patients were treated within 3–8 h of acute spinal injury, and the study maintained the bolus dose of 30 mg/kg that was used in NASCIS 2 [86]. However, half of the participants received the 5.4 mg/kg/h methylprednisolone infusion for a total of 24 h and half of the participants received the infusion for 48 h. Neurologic recovery was measured and patients were followed for 6 months. The study results confirmed the neurologic benefit of early megadose corticosteroids following acute spinal injury. The study concluded that 48 h treatment benefits patients, if treatment is initiated 3–8 h after injury. This group had improved motor recovery and self-reported functional independence with 48 h steroid therapy. However, there was also an increased likelihood of severe sepsis and severe pneumonia in the 48 hour steroid infusion group. For patients who were treated within 3 h of injury, there was no significant difference between 24 and 48 h treatment regimens, and it was advised to continue using 24 h therapy for this group to reduce the complications of steroids [86].
Following the NASCIS 2 and 3 reports of neurologic recovery with megadose corticosteroids, researchers have sought to apply the results to patients with indirect TON [8]. An increasing number of patients with TON were managed with high-dose corticosteroids [10]. The results of research studies have varied widely. Several studies found a positive relationship between high-dose corticosteroids and recovery from TON [14, 44]. However, some studies comparing corticosteroid managed and conservatively managed patients with TON did not yield significantly different visual outcomes [29, 43]. There were also studies comparing corticosteroid management with surgical management that did not find a difference in treatment approach [62].
The research regarding indirect TON treatment has been limited by several factors. Many studies had small sample sizes or a lack of control group. Ability to compare studies has been greatly limited [10, 43]. Studies differed on multiple factors including differences in the timeline of treatment, corticosteroid dosing, baseline visual acuities, follow-up frequency, inclusion criteria, and exclusion criteria. Furthermore, in several studies, patients received combined corticosteroid and surgical treatment, which made it difficult to study the efficacy of each individual treatment [42, 73].
In 1999, the International Optic Nerve Trauma Study (IONTS) was established to address the limitations of indirect TON treatment research in evaluating the effect of corticosteroid treatment [28]. The study was designed as a randomized controlled trial, but was later modified to an observational nonrandomized study due to difficulties with patient recruitment. The study followed three types of treatment groups: corticosteroid only, surgical decompression only, and conservative management. In the corticosteroid treatment group, 40% received megadose steroids consistent with the NASCIS trials, while 60% received lower doses (see Table 12.4). For all treatment groups, the initial visual exam was conducted within 3 days of injury and treatment was initiated within 7 days of injury. Visual outcomes were assessed by change in VA. After adjustment for baseline visual acuity, the researchers concluded that there was no difference in visual outcome between the treatment groups [28].
Corticosteroid dose (mg/day) | Percentage patients in IONTS | |
---|---|---|
Megadose | >5400 | 40 |
Very high dose | 2000–5399 | 18 |
High dose | 500–1999 | 19 |
Moderate dose | 100–499 | 9 |
Low dose | <100 | 6 |
In addition, the researchers did not find that timing or dose of treatment had an effect on the visual outcome. The researchers concluded that the the type of treatment should be made on a case-by-case basis, and there is no clear evidence to recommend one treatment over another [28].
The IONTS has merit as the first prospective study to evaluate the treatment of indirect TON. The researchers used a standardized method of defining and comparing visual improvement between the treatment groups [28]. The IONTS was a large patient study, and patient enrollment was great enough to detect statistical differences between the corticosteroid and surgical treatment groups [9]. However, there were study limitations. There were fewer patients in the conservative management group, and the conclusions regarding this group do not have as much power. Furthermore, the study design was not randomized, controlled, or masked. Because it became an observational study, there may have been selection bias regarding choice of treatment, and some patients were treated with both corticosteroids and surgery [8]. There were differences in corticosteroid dose, timing of treatment, and criteria for optic canal decompression between the patients [11].
Since the IONTS, corticosteroid research for indirect TON remains limited. The 2013 Cochrane Eyes and Vision Group review of steroids for TON identified only one randomized double-blind controlled trial to address the use of high-dose corticosteroids for indirect TON [20]. The research, by Entezari et al. in 2007, randomized patients with indirect TON to either a high-dose corticosteroid group (1000 mg methylprednisolone/day over 72 h) or a placebo group [58]. Patients were diagnosed with indirect TON within 7 days of injury based on clinical presentation of RAPD and decreased visual acuity. On average, they were treated within 52 h of injury. The patients were followed for 3 months and visual improvement was measured by visual acuity. At 3 months, there was no significant difference in visual recovery between the treatment and control groups. This conclusion supports the results of IONTS.
In addition to questions about corticosteroid efficacy, there have been concerns about treatment risks [63]. NASCIS 2 identified more frequent gastrointestinal bleeds in the steroid-treated group, and NASCIS 3 identified more severe sepsis and pneumonia in the 48-hour steroid-treated group [85, 86]. In addition, wound infections, pancreatitis, and acute psychosis have been reported following high doses of methylprednisolone [11].
In 2004, the Corticosteroid Randomization After Significant Head Injury (CRASH) trial investigated the safety of megadose corticosteroids administered for head injury [87]. The CRASH study was an international, multicenter, double-blind randomized placebo-controlled trial that enrolled 10,008 patients with head trauma. The patients were randomized within 8 h of injury. One group received a 2 g methylprednisolone over 1 hour in a 100 mL infusion, followed by a maintenance dose of 0.4 g/h for 48 h in a 20 mL/h infusion and the other group received a placebo. The doses were set to correspond with the NASCIS 3 trial. The study recruitment was stopped early when unmasked results indicated higher mortality rates in the steroid-treated group. At 2-week follow-up, there was a significantly greater risk of death from all causes for the patients in the corticosteroid group [87]. At 6-month follow-up, the researchers found a significantly higher risk of mortality and severe disability in the steroid-treated group [88]. This effect did not change based on injury severity or time from injury. The researchers concluded that corticosteroids are not recommended for cases of head trauma. The study results can extrapolate to the treatment of indirect TON because many of these patients also experienced head trauma [8].
Concerns about corticosteroid risk have been substantiated by animal studies. Steinsapir and coworkers examined the effect of very high-dose corticosteroids on injured optic nerves in rats [89]. Following crush injuries to the optic nerve, rats either received very high-dose methylprednisolone or saline placebo. When the optic nerves were examined six weeks after crush injury, the rats that underwent steroid treatment lost more axons than the placebo group. The study indicates that methylprednisolone may be directly toxic to a damaged optic nerve.
Further research with double-blind RCTs is needed to elucidate how steroids should be used in the treatment of indirect TON. Based on the IONTS and the study by Entezari et al., there is no clear evidence that steroids provide an additional benefit in the treatment of indirect TON. Future research regarding other doses and timeframes of corticosteroid treatment may alter these conclusions. Given the CRASH trial results, steroids should not be given to patients with head injuries. Other patients should be carefully evaluated on a case-by-case basis.
Surgical management
Surgical management of indirect TON is focused on reducing secondary injury to the optic nerve (See: Pathophysiology section). Optic nerve edema primarily affects the intracanalicular segment of the optic nerve. Optic canal decompression relieves the secondary intracanalicular compartment syndrome by providing more space for the edematous optic nerve and limiting post-injury ischemia [8, 11, 29, 90]. Early surgical intervention is believed to augment the potential for visual recovery [19, 91].
Surgical indications for TON are limited by research studies, which are primarily small and retrospective [19, 23]. The criteria are well defined for optic nerve hematoma, but surgical indications are less clear with optic canal fracture or indirect TON.
There is strong evidence to support surgical management in cases of hematoma (See: Direct TON-hematoma section). With retrobulbar hematoma or optic nerve sheath hematoma, urgent surgical decompression and evacuation of the hematoma is indicated [11, 58, 63]. Surgical evacuation of hematoma with optic nerve sheath fenestration for intrasheath hemorrhage quickly relieves optic nerve compression [24]. In case reports of patients with radiological evidence of enlarged optic nerve sheaths and gradual visual loss, there is a positive response to optic nerve sheath decompression with a high potential for visual recovery [8].
With optic canal fracture, surgical management with optic nerve decompression has been recommended. In particular, surgery has been recommended for comminuted canal fracture or radiologic evidence that the fractured optic canal segment impinges on the optic nerve [11, 19, 24, 28, 45, 58]. However, optic canal fracture has been identified as a poor prognostic factor, regardless of intervention, and researchers question if surgery is beneficial in all cases of optic canal fracture [20]. Poor outcome is related to optic nerve transection. If the bone fragments transected the optic nerve, the injury to RGCs is irreversible and optic canal decompression would not have an effect on visual function [19]. As a result, case studies indicate that surgical decompression for patients with optic canal fractures has low potential for significant visual improvement [92].
The considerations for indirect TON are not as well defined. Given the pathophysiology of indirect TON, optic nerve swelling is a commonly identified reason for optic canal decompression [8]. Intraneural edema, diagnosed radiologically and suggestive of indirect TON, has been cited as an indication for surgical management [11].
Surgical candidates are identified based on the likehood of visual recovery. Indirect TON patients with gradual visual decline and VA better than NLP are considered the best candidates for surgical decompression [49, 93]. Gradual visual change following injury is considered to indicate a reversible cause of vision loss, whereas sudden onset, acute and complete vision loss is more likely to indicate a nonreversible process such as optic nerve transection [14]. Given their poor prognosis, it is unclear if there is a benefit to treating patients with total, sudden vision loss following trauma [42, 94]. In retrospective studies, few patients (0–17%) with sudden vision loss who were NLP at presentation regained vision after surgical decompression [14, 95]. In comparison, the same retrospective studies indicated that the majority of patients with vision better than NLP experienced some degree of visual recovery either after surgical decompression or conservative management [95].
Because the guidelines for optic canal decompression are unclear, the surgery should only be considered for conscious patients [15, 83]. These patients are able to undergo a full ophthalmic exam to first rule out other causes of visual loss [42]. They can also be counseled regarding the risks and benefits of surgery, and actively participate in the decision-making process [10, 90].
It is widely understood that earlier management of indirect TON yields better visual outcomes. Several reports define effective surgical management as occurring within 1 week of trauma [15, 49, 60, 96].
High-resolution CT scans of the intraorbital and intracanalicular optic nerve are helpful to visualize optic nerve pathology and evaluate the need for surgical management [49]. CT findings that indicate a need for surgical management include an enlarged optic nerve sheath suggestive of hemorrhage, optic nerve edema, or optic canal fracture [42, 49]. After surgical need is identified, CT scans are further utilized in surgical planning [42]. For example, imaging the orbital apex identifies the relationship of the optic nerve and the carotid artery, which is critical to reduce intraoperative complications [90].
There are a variety of surgical techniques for optic nerve decompression. In all approaches, a wall of the optic canal is removed to provide room for the edematous optic nerve [45]. The earliest technique for optic canal decompression, developed in the 1960s, takes an intracranial approach to reach the optic canal [15, 92, 97]. With a craniotomy, surgeons are able to directly visualize a large area of the brain and potentially discover coexistent areas of brain damage following trauma [45]. However, the intracranial approach has significant risks to the patient and outcomes are overall poor [14, 49]. It is an invasive procedure and requires an external incision. Possible consequences of intracranial optic nerve decompression include frontal lobe edema due to the need to retract the frontal lobe, and the loss of olfaction [91, 94, 96].
Extracranial approaches to reach the optic canal were developed as a less invasive way to achieve optic canal decompression [49]. There are a variety of extracranial approaches, and all surgical techniques have the same final step of removing a wall of the optic canal to achieve decompression. The extracranial transethmoidal approach is most frequently used [10, 42]. Approaches that have been reported include transconjunctival [73, 98], transantral [92], transethmoidal [60, 92, 99, 100], transnasal [10], and lateral facial [101]. Combined techniques include transethmoidal/transorbital [90], transethmoidal/transsphenoidal [91], endonasal transsphenoidal [96, 102–104] and transconjunctival/intranasal endoscopic approaches [94].
Extracranial approaches offer several advantages over intracranial optic canal decompression. Because the approach is less invasive, it can be completed under general anesthesia [45, 92]. There is no external incision, no risk of frontal lobe edema or decreased olfaction, a faster recovery time, and decreased morbidity compared to intracranial techniques [91, 94, 96].
Although safer than intracranial surgery, extracranial optic canal decompression has several reported complications. All of the extracranial approaches risk cerebrospinal fluid leak, meningitis, intraorbital infections, and accidental dural exposure [11, 23, 91]. For example, in the IONTS, 3 out of 33 patients treated with extracranial decompression experienced CSF leak, and one developed meningitis postoperatively [28]. Enophthalmos may result from expansion of orbital volume [90, 94].
There is risk of damage to the surrounding anatomy in the orbit and the sellar region [9]. Direct, thermal, or ischemic injury to the optic nerve can occur intra-operatively [14, 90, 105]. There may be injury to the lacrimal apparatus, ophthalmic artery, or surrounding brain tissue [11, 91, 92, 94]. With a transconjunctival approach, there is risk for medial rectus entrapment and injury to the globe [94]. All approaches to optic canal decompression carry the serious risk of injury to the carotid artery because the optic nerve travels close to the carotid artery near the sphenoid sinus [10]. There is high mortality associated with damage to the carotid artery due to difficulty controlling hemorrhage, subsequent intracerebral hypoperfusion, and extravascular dissection of the expanding hematoma [90]. If carotid artery laceration occurs, immediate management should include sinus pressure packing tamponade, carotid artery ligation, and possible endovascular tamponade [90].
Ultimately, selection of surgical method should be individualized. Surgeon experience, location of pathology, and patient anatomy should all be considered. The intracranial approach still has use if intracranial pathology secondary to trauma also needs to be addressed [90]. When pathology is localized to the optic nerve, an extracranial approach is safer for the patient.
As with corticosteroid treatment, the decision to manage indirect TON with surgical decompression has not been standardized and the choice to perform surgery should also be made on an individual basis. Several studies have reported success with optic nerve decompression; however, these are frequently uncontrolled, small, retrospective case studies so it is difficult to make clear conclusions about surgical indications or timing [49, 60]. Furthermore, it is not possible to compare published studies because a variety of intracranial and extracranial surgical decompression techniques are employed, and frequently corticosteroid treatment is given to surgical patients in non-standardized regimens [11, 91]. There is also a recruitment bias prevalent in the literature, with selection of patients who have worse baseline visual acuities or have already failed corticosteroid treatment [11, 12]. Based on a Cochrane Review examining Optic Nerve Decompression for TON, there are no randomized controlled trials in which any form of surgical intervention for TON, either on its own or in combination with steroids, was compared to steroids alone or no treatment [23]. The IONTS (see: Corticosteroid Treatment section) could not find a reason to recommend surgical management for indirect TON over corticosteroids or conservative management [62]. Without clear evidence that surgical decompression benefits patients with TON, and given the well-known risk factors of the surgery, the decision to perform optic nerve decompression will remain controversial until further research is conducted [23].
Other Treatment Strategies
The goal of experimental treatment studies for TON is neuroprotection. Following trauma, irreversibly damaged RGCs release neurotransmitters and inflammatory factors that induce apoptosis of nearby, undamaged RGCs. New treatment strategies aim to prevent the receptive secondary apoptosis and minimize total damage to the ON [11, 19, 83]. For example, glutamate inhibitors prevent apoptosis in animal models [106]. Neurotrophic factors including fibroblast growth factor [2, 107], brain-derived neurotrophic factor [108, 109], and ciliary neurotrophic factor [110, 111] combat growth factor deficiencies following TON and promote survival of remaining RGCs. Additional neuroprotective research includes the anti-inflammatory small heat-shock protein crystalline [112], nitric oxide synthase inhibitors [113], tumor necrosis factor-alpha inhibitors [114], calcium channel blockers [115], the immunosuppressant FK50 [6, 116], and erythropoietin [117]. These interventions are promising, but still in early stages of investigation.
Traumatic Pupillary Abnormalities
Two significant types of traumatic pupillary abnormalities are Adie’s tonic pupil, a dysfunction of the ciliary ganglion, and Horner syndrome, which involves dysfunction of sympathetic pathways of the nervous system. Both disorders produce characteristic changes in the pupil that are described below.
Adie’s Tonic Pupil
Adie’s tonic pupil results when postganglionic parasympathetic nerves to the iris sphincter and ciliary muscle are lost. The loss of this innervation results in a fixed dilated pupil that reacts poorly to light, but will constrict to near stimuli. When the near stimuli are removed, the characteristic tonicity becomes apparent as the pupil slowly relaxes. Additionally, Adie’s tonic pupil is known to be supersensitive to weak miotic agents such as pilocarpine due to cholinergic denervation [118]. Adie’s pupil can be associated with findings such as decreased deep tendon reflexes and the presence of anhidrosis. This triad of findings is known as Ross syndrome [119].
Adie’s tonic pupil is often idiopathic. Women are more often affected with a mean age of 32 years [120]. The tonic pupil initially presents unilaterally in 80% of cases, and the chance of second pupil involvement is 4% per year [120]. Underlying causes that may be responsible for the parasympathetic denervation include traumatic, iatrogenic, viral, and neoplastic etiologies.
Horner Syndrome
Horner syndrome involves disruption of the sympathetic efferent fibers that normally cause the pupil to dilate, assist the lid in retraction, and enable perspiration of the forehead. The sympathetic pathway involved begins in the hypothalamus and, over the course of three neuron synapses, descends down the cervical cord, exits through the ventral rami of C8, T1, and T2, and joins the paraverterbral sympathetic chain to ascend some levels before continuing along the wall of the carotid artery and exiting the carotid wall to innervate its targets [121]. Dysfunction along the pathway, as depicted in Fig. 12.17, results in the typical findings of Horner syndrome: ptosis, miosis, and anhidrosis.


Fig. 12.17
Three-neuron sympathetic innervation of the eye. AS ansa subclavia; ECA external carotid artery; ICA internal carotid artery; ICG inferior cervical ganglion; MCG middle cervical ganglion; SCG superior cervical ganglion; FON first-order neuron; SON second-order neuron; TON third-order neuron. From Reede D, Garcon E, Smoker W, Kardon R. Horner’s Syndrome: Clinical and Radiographic Evaluation. Neuroimaging Clinics of North America [serial online]. January 1, 2008;18(Cranial Nerves):369–385. Available from: ScienceDirect, Ipswich, MA. Reproduced with permission from Science Direct
Epidemiology
Spontaneous internal carotid artery dissection has been reported to present as Horner syndrome in approximately 28–41% of cases [122]. Internal carotid artery dissection occurs with an incidence of 2.6–3.0 in 100,000, and with equal occurrence rates in males and females, most often of young and middle-ages [123].
In one study of combat-associated ocular trauma, Horner syndrome due to upper chest and neck injury represented approximately 1% of the total cases. Similarly, a series of TBI patients demonstrated Horner syndrome in 0.9% of cases [124]. In one rare case, Horner syndrome was reported as a direct consequence of first rib fracture, itself a rare occurrence, suffered in a motor vehicle accident without carotid dissection [125].
Iatrogenic Horner syndrome can be a rare complication of different surgical procedures involving the head and neck, as described below. Even with closely associated procedures, such as conventional thyroidectomy, the occurrence of Horner syndrome has been reported to be as low as 18 cases between 1993 and 2015 [126].
Pediatric Horner syndrome, although rare, can be acquired or congenital. One study determined the pediatric incidence to be 1.42 per 100,000 patients younger than 19 years, and congenital syndrome to occur in 1 in 6250 births [127]. In a series of 73 pediatric patients with Horner syndrome, 42% were considered congenital or caused by birth trauma, 15% were acquired without surgical intervention such as by neoplasm or trauma, and 42% were acquired with surgical intervention as the cause [128].
Presentation
Horner syndrome consists of the three classic findings of ptosis, miosis, and anhidrosis. Though these three findings make up the syndrome, dysfunction along the third-order, postganglionic neuronal fibers that run along the internal carotid artery will involve only the fibers responsible for mydriasis and lid retraction, thus resulting in a Horner syndrome with spared facial sweating [123].
In Horner syndrome caused by carotid artery dissection, common symptoms include ipsilateral headache in 68–92% of cases, focal cerebral deficits in 49–67% of cases, and cranial nerve palsies in 12–14% of cases [123].
Pathophysiology
Often, the syndrome occurs due to cervical-carotid dissection that can be caused by a motor vehicle accident (MVA), penetrating trauma to the upper chest or neck or iatrogenically [122, 129]. Such damage to the motor neuron pathway along its anatomical course will result in the signs and symptoms of Horner syndrome.
Iatrogenic Horner syndrome occurs with multiple types of procedures due to the extensive pathway of the sympathetic system along the spinal cord and carotid artery. Procedures involving manipulation of the neck, such as in chiropractic manipulation or endotracheal tube placement have the risk of causing iatrogenic Horner syndrome. The association with thyroid surgery described above is thought to be due to the contiguous localization of the oculosympathetic ganglion with neurovascular supply to the thyroid gland, complicating the isolation of thyroid structures during removal without causing Horner syndrome [126]. Other possible etiologies include postoperative hematoma compressing the sympathetic pathway, ischemia to the neurons, and stretching during retraction [130]. Minimally invasive video-assisted thyroidectomy is also thought to be associated with the occurrence of Horner syndrome, due to the limited mobility afforded to the endoscopic tools used in the procedure by the region of anatomy [126].
One example of surgical intervention resulting in a pediatric case of Horner syndrome is from a case report of pulmonary hydatid cyst removal in a 10-year-old girl whose ptosis recovered in 3 months and anisocoria recovered in 6 months [131].
Treatment
Treatment of Horner syndrome secondary to cervical-carotid dissection with antiplatelet or anticoagulant medication can reduce the risk of stroke. Often, heparin bridged with warfarin to an INR of 2.0–3.0 for 3–6 months is used until the dissection resolves of its own accord, which occurs in 85–90% of cases [123]. Antiplatelet drugs such as aspirin or clopidogrel are also used frequently, either alone or in combination [132]. Between the two therapies, a recent randomized controlled trial of 250 patients with carotid or vertebral dissection determined that the recurrence of stroke on either therapy at 3 months was lower than found in previous studies, and that there was no significant difference between antiplatelet or anticoagulant medication in regards to the rare outcomes of recurrent ipsilateral stroke or death [132]. Patients who fail medical management of carotid artery dissection may be closely monitored or undergo surgical intervention, such as intravascular stenting [123].
Head Injury
In the United States, head trauma is a frequent cause of emergency room visits. In 2013, the American College of Surgeons’ National Trauma Data Bank reported that 36% of adult trauma admissions involved head injury [133]. Typically, the demographic of head trauma patients is composed of young males [134]. Head injuries are most commonly caused by motor vehicle accidents, and non-restrained passengers have a greater severity of injury [2, 50, 135]. Patients with the most severe ocular injury often present with a GCS score less than or equal to eight [2, 135]. Patients who lose consciousness following closed head injury are more likely to have permanent neurologic injury [50].
A variety of neuro-ophthalmic injuries can result from closed head trauma. In a retrospective review of 181, patients with closed head trauma and no preexisting ocular disease, primary ophthalmic complaints included: blurred or decreased vision (46%), diplopia (30%), headaches (13%), trouble reading (6%), ocular irritation (3%), oscillopsia (2%), pupil irregularity (2%), sagging eyelids (2%), and photopsia (2%) [50]. Constricted visual field loss was most frequently observed (41% of reported visual field defects), but any part of the visual pathway may be injured [50].
In the evaluation of head injury and its visual complications, the ophthalmologist should consider skull base fractures, intracranial contusions, arterial or venous injury, direct penetrating trauma to visual pathways, and TBI.
Skull Base Fractures
Epidemiology and Mechanism
A skull base fracture extends through any of the cranial floor bones: the orbital plate of the paired frontal bones, cribriform plate of the ethmoid bone, occipital bone, sphenoid bone, and petrous or squamous portions of the paired temporal bones [133, 136]. Skull base fractures are most often caused by high impact, accidental closed head trauma [137]. Approximately 7–16% of all closed head trauma result in basilar skull fracture [133]. Iatrogenic injury during skull base surgery is a less frequent cause of skull base fracture.
Accidental Trauma
Blunt head trauma causes over 90% of skull base fractures [137]. Motor vehicle accidents are the most frequently reported mechanisms, often involving unrestrained passengers in high-speed collisions [138, 139]. In a review of 81 cases of basilar skull fracture, 59% of patients were in vehicular accidents, 35% were injured by high velocity falls from a height, and 6% were assaulted [140]. These high impact mechanisms of skull base fracture are consistently cited throughout the literature [133]. The majority of patients are young and male [140].
Penetrating trauma, such as injury from a gunshot, is a less common mechanism of skull base fracture [133, 137]. Skull base fractures from assault with projectile weapons have increasing incidence in urban settings [141].
The force and direction of impact predicts the type of skull base fracture pattern and associated complications. Most commonly, a fracture involves the anterior skull base and is associated with direct, frontal impact [133]. A high velocity, lateral force of impact may produce a fracture that extends from the anterior skull base to the middle and posterior skull base, or a fracture in the coronal plane through the central skull base [133, 138, 142]. Isolated fractures of the posterior skull base are commonly from lateral or posterior head injury [133].
Because surrounding bony structures heavily protect the skull base, significant trauma is needed to produce a skull base fracture [141]. Such large-force trauma is likely to present with multiple injuries. Complex facial or orbital fractures are often comorbid with basilar skull fracture [133, 137].
Iatrogenic Trauma
Iatrogenic skull base fracture is most frequently associated with complications of functional endoscopic sinus surgery (FESS) [143, 144]. Less than 1% of FESS are complicated by skull base injury with CSF leak [144]. The skull base damage most often affects the lateral lamella of the cribriform plate or the posterior ethmoid roof [143].
Additional procedures that have been implicated in skull base fracture are septoplasty, otologic surgery including removal of vestibular schwannoma, transsphenoidal pituitary resection, craniofacial resection, and transcranial approaches to access the optic chiasm or cavernous sinus [143, 145, 146].
Anatomy of the Skull Base and Clinical Implications in Skull Base Fracture
The skull base forms the floor of the anterior, middle, and posterior cranial fossae. The contents of each fossa have important clinical implications in skull base fracture (see Table 12.5: skull base openings and their contents, and Figs. 12.18 and 12.19). The clinical presentation can vary greatly based on the location and severity of the fracture [137]. Patients with basilar skull fracture should be evaluated for dural laceration with CSF leak, infection, contusions, pneumocephalus, brain laceration, encephalocele, meningoencephalocele, intracranial hematomas, cranial nerve palsy, and neurovascular injuries [50, 133, 136, 141, 147].


Table 12.5
Skull base openings and their contents
Location in skull base | Opening | Contents |
---|---|---|
Anterior | Cribriform plate | Branches of olfactory n. (CN I) |
Middle | Optic canal | Optic n. (CN II), ophthalmic a. |
Superior orbital fissure | Oculomotor n. (CN III), trochlear n. (CN IV), ophthalmic division of trigeminal n. (CN V1), abducens n. (CN VI), superior ophthalmic v. | |
Foramen rotundum | Maxillary division of trigeminal n. (CN V2) | |
Foramen ovale | Mandibular division of trigeminal n. (CN V3), accessory meningeal a., lesser superficial petrosal n., emissary veins | |
Foramen spinosum | Middle meningeal a. (branch of external carotid a.), meningeal branch of CN VII | |
Foramen lacerum | A. of the pterygoid canal, n. of pterygoid canal, venous drainage | |
Carotid canal | Internal carotid a., sympathetic plexus | |
Posterior | Internal acoustic meatus | Facial n. (CN VII), vestibulocochlear n. (CN VIII), labyrinthine a. and v. |
Jugular foramen | Internal jugular v., glossopharyngeal n. (CN IX), vagus n. (CN X), accessory n. (CN XI), inferior petrosal sinus, posterior meningeal a. | |
Hypoglossal canal | Hypoglossal n. (CN XII), venous plexus of hypoglossal canal | |
Foramen magnum | Spinal v., anterior spinal a., posterior spinal a., spinal cord, accessory n. (CN XI), vertebral a. |

Fig. 12.18
Anterior, middle, and posterior cranial fossae. From Baugnon and Hudgins [133]. Reproduced with permission from Elsevier

Fig. 12.19
Skull base anatomy. From BRS Gross Anatomy, 7th edition. Reproduced with permission from Wolters Kluwer
For a summary of skull base fracture locations and complications, see Table 12.6.
Table 12.6
Location of skull base fracture and frequently associated complications
Location | Complication |
---|---|
Anterior skull base | Anosmia (CN I injury) Intraorbital injury CSF rhinorrhea/meningoencephalocele |
Middle skull base | Vascular injury: ICA occlusion, dissection, pseudoaneurysm, aneurysm, carotid-cavernous fistula CN injuries: II, III, IV, V, VI Horner’s syndrome |
Posterolateral skull base (temporal bone) | Vascular injury: ICA CN injuries: VII, VIII CSF otorrhea/meningoencephalocele |
Posterior skull base | Venous vascular injury or vertebrobasilar injury CN injuries: IX, X, XI, or XII Craniocervical junction and cervical spine injuries |
Anterior Skull Base Fracture
The anterior skull base, formed by the orbital portion of the frontal bones and the ethmoid bone, is the most common site of basilar skull fracture [138]. The anterior skull base begins at the posterior wall of the frontal sinus and extends posteriorly to the anterior clinoid processes and planum sphenoidale. Due to its location and structure, the anterior cranial fossa absorbs extrinsic force directed to the mid-facial skeleton or anterior cranial vault. It limits energy transfer and damage of the more posterior cranial contents [138].
The olfactory nerve fibers pass through the thin cribriform plate of the ethmoid bone, and are commonly disrupted with traumatic anterior skull base fracture or during transethmoidal surgical approaches to the anterior skull base [148]. Patients with anterior skull base fracture can present with anosmia due to olfactory nerve damage, epistaxis from damage to anterior and posterior ethmoidal arteries, or frontal lobe contusion [133, 137].
There is a close spatial relationship of the anterior skull base with the paranasal sinuses and the orbit. Given the high impact trauma needed to cause anterior skull base fracture, the paranasal sinuses and orbit may also be injured [133, 141]. If there is damage to the optic nerve or intraorbital structures, patients may have decreased visual acuity, proptosis, enophthalmos, orbital hematoma, diplopia, chemosis, increased intraocular pressure, or other visual findings [137].
High impact trauma can cause meningeal tears, and closed head injury is the most common etiology of CSF leaks [136, 143]. Traumatic CSF leak occurs in 10–45% of skull fractures [136, 139, 140, 149]. In the majority of cases, CSF fistula manifests as CSF rhinorrhea, in which a meningeal tear results in a communication between the intracranial and nasal cavities [133, 137, 140, 149]. CSF rhinorrhea is most often caused by anterior skull base fracture, and less often by temporal bone fractures [149]. 80% of patients with CSF rhinorrhea following basilar skull fracture present within 48 h after trauma, and about 95% of cases are evident by the first 3 months of injury [133].
CSF leak poses a risk for meningitis due to communication of the disrupted meninges with the flora of the nasal or middle ear cavities [133, 136]. Meningitis has been reported in about 10–15% of patients with CSF rhinorrhea [149]. The risk of meningitis increases with time. There is a 1% risk of meningitis in the first 24 h after injury with CSF fistula. The meningitis risk increases to 9% after one week and 18% after 2 weeks [133, 149].
CSF hypotension is a complication of chronic CSF leak. The patient may have optic disc edema, enophthalmos, diplopia, orthostatic headaches, posterior neck pain, nausea, or vomiting. CSF may be identified on MRI by subdural fluid collections, pachymeningeal enhancement, venous engorgement, pituitary hyperemia, and downward displacement of the brain [150]. CSF hypotension has an opening pressure less than 60 mmHg.
Middle Skull Base Fracture
The middle skull base begins anteriorly at the greater wing of the sphenoid bone and ends posteriorly at the clivus. The greater wing of the sphenoid bone forms the anterior floor of the middle skull base, and the anterior petrous bone forms the posterior floor. The middle skull base has many important structures that are susceptible following fracture (see Table 12.5: skull base openings and their contents). Vascular complications are most frequently reported following middle skull base fracture. In particular, the internal carotid artery is susceptible due to its course in the cavernous sinus and carotid canal of the petrous bone.
Middle skull base fractures occur from high impact injury, and there are often serious associated intracranial injuries, such as DAI (see: Traumatic Brain Injury section) or multi-compartmental hemorrhage. The temporal lobe in the middle cranial fossa is susceptible to contusion injuries [148].
Optic Canal
The optic nerves exit the orbit through the optic canals, which pass through the lesser wing of the sphenoid bone. The anterior clinoid processes form the roof of the optic canal. Compression of the optic nerve from middle skull base fractures may result in decreased visual acuity and a RAPD (see: Section “Optic Nerve Trauma”).
Superior Orbital Fissure
The superior orbital fissure is the space between the lesser and greater wings of the sphenoid bone. Multiple cranial nerves (III, IV, V1, VI) and the superior ophthalmic vein pass through the superior orbital fissure and may be injured in middle skull base fracture. CN III, IV, and VI palsies lead to various degrees of ophthalmoplegia (see: Section “Oculomotor System”).
Other important foramina in the middle skull base are the foramen rotundum, foramen ovale, and the foramen spinosum. The foramen rotundum is posterior and inferior to the base of the superior orbital fissure and transmits the maxillary division of the trigeminal nerve (V2). The foramen ovale is posterior and lateral to the foramen rotundum and transmits the mandibular division of the trigeminal nerve (V3). The foramen spinosum is further posterior and lateral and transmits the middle meningeal artery.
Sella Turcica
The sella turcica of the sphenoid bone is in the central part of the middle skull base. It is located posterior and medial to the two orbits, between the anterior and posterior clinoid processes. The hypophyseal fossa, a depression within the body of the sphenoid bone, holds the pituitary gland.
The optic chiasm is inferior to the sella turcica. Due to its anatomical relationship, fractures of the sella turcica can result in chiasmatic compression, laceration, contusion, hematoma, or ischemic compromise [133, 151]. Traumatic chiasmal syndrome describes the consequences of sagittal midline skull base fractures through the sella turcica. Patients have complete or partial bitemporal hemianopsia that is consistent with optic chiasm injury [152]. Optic chiasm lesions are rare, and have been observed in only 4.4% of patients with visual complications following head trauma [153]. It is best diagnosed by MRI [154]. Additional neurologic features observed in traumatic chiasmal syndrome are diabetes insipidus from interruption of the hypothalamic-pituitary apparatus, panhypopituitarism, intrasellar hematoma, pneumatocele, or cranial nerve defects [151–153]
Internal Carotid Artery
The internal carotid artery (ICA) course has four parts: cervical (ascending through the neck), petrous (crossing the temporal bone), cavernous, and cerebral (see Fig. 12.20). The cervical part ascends the neck in the carotid sheath without any branches, and enters the petrous bone through the carotid canal. The petrous part gives off the caroticotympanic and pterygoid branches. It travels along the petrous bone and then enters the cavernous sinus between the anterior and middle clinoid processes, medial to the abducens nerve. The cavernous part has small branches to the wall of the cavernous sinus, hypophysis, and the semilunar ganglion of the trigeminal nerve. In its last segment, the ICA enters the middle cranial fossa and branches into the ophthalmic artery, anterior choroidal artery, and posterior communicating artery (PcommA). It finally bifurcates into the anterior and middle cerebral arteries, and these terminal branches form part of the Circle of Willis (See: Vascular Trauma).
Fig. 12.20
Course of the internal carotid artery, as demonstrated with an arterial phase of a right internal carotid angiogram, lateral view. Nolte [168], 6th Ed. Reproduced with permission from Elsevier
The cavernous and petrous segments of the ICA are most susceptible to basilar skull fracture due to proximity to the skull base. ICA injury carries severe risk of stroke, pseudoaneurysm, and death [137].
The ICA carries postganglionic sympathetic fibers. If the postganglionic sympathetic fibers traveling on the ICA are injured, patients can develop partial Horner Syndrome with miosis and ptosis [133]. Anhidrosis is an unlikely manifestation of Horner syndrome from middle skull base fracture, because sympathetic nerve fibers responsible for sweating travel separately with the external carotid and maxillary arteries [155].
Cavernous Sinus
The cavernous sinus is a paired structure located within the sphenoid bone on either side of the sella turcica. It contains CN III, IV, V1, V2, VI, the cavernous portion of internal carotid artery, and postganglionic sympathetics traveling on the ICA (see Fig. 12.21). Cranial nerve neuropathies may follow trauma involving the cavernous sinus [133]. CN VI is most susceptible due to its medial location in the cavernous sinus. Comparatively, CN III, IV, V1 and V2 are located in the lateral cavernous sinus, where the dural attachments of the cavernous sinus are protective [156].
Fig. 12.21
Cavernous sinus anatomy. From Moore Clinically Oriented Anatomy. 6th Ed. Reproduced with permission from Wolters Kluwer
The cavernous sinus has multiple venous connections, creating an overall low-pressure venous system (see Fig. 12.22) [156]. The anterior and posterior intercavernous sinuses create a communication between the right and left cavernous sinuses. Anteriorly, the superior and inferior ophthalmic veins and the sphenoparietal sinuses drain into the cavernous sinus. Posteriorly, the cavernous sinus drains into the superior and inferior petrosal sinuses, which will ultimately drain into the sigmoid sinus, transverse sinus, and internal jugular vein. Superiorly, the superficial, middle, and inferior cerebral veins approach the cavernous sinus. Inferior to the cavernous sinus, the emissary veins drain into the pterygoid plexus. If there is an ICA injury in the region of the cavernous sinus, there is risk of carotid-cavernous fistula (CCF) formation with backup of blood into the venous system (see carotid-cavernous fistula section later in this chapter) [137, 157].
Fig. 12.22
Anatomy of the cavernous sinus drainage system. From BCSC Neuro-ophthalmology. Reproduced with permission from American Academy of Ophthalmology
Petrous bone
The petrous bone is commonly involved in middle skull base fractures [137]. The most frequent clinical presentation of petrous bone fracture is conductive hearing loss due to middle ear and ossicular chain damage [137]. Patients may also have postauricular hematoma (Battle’s sign), hemotympanum, or CSF otorrhea. There may be vascular injury to the ICA or to the superior petrosal sinus, which courses along the medial border of the petrous bone in the petrous ridge [148].
There may be associated cranial nerve injury, especially sensorineural deafness, vertigo, or tinnitus from CN VIII injury or facial palsy from CN VII injury. CN VI palsy is less common with middle skull base fracture, but it can occur if the middle skull base fracture extends to the petrous apex and sphenoid sinus [155]. Such an extensive fracture would produce traumatic petrous apex syndrome, involving CN V, VI, VII, and VIII. The CN VI palsy is often caused by benign stretching injury and usually spontaneously resolves in a few months (See: Oculomotor Injury Section) [158].
Posterior Skull Base Fracture
The posterior skull base begins anteriorly at the clivus and it is formed by the occipital bone and the posterior temporal bone. The posterior cranial fossa holds the cerebellar hemispheres, midbrain, pons, and medulla. The cerebellum is separated from the temporal and occipital lobes by the tentorium cerebelli.
The posterior skull base is the least likely location for skull base fracture. The majority of posterior skull base fractures involve the petrous and occipital bones [133, 137]. The severity of traumatic impact and coup-contrecoup injury may cause parieto-occipital contusions and associated visual field defects [50].
Epidural hematoma (EDH) is the most common complication seen with posterior skull base fractures. The venous sinuses, particularly the sigmoid sinus, jugular bulb, and transverse sinus, are susceptible in this region [133]. If a posterior skull base fracture lacerates the posterior venous sinuses, it can cause a posterior fossa venous EDH [137]. There is high risk of rapid hematoma expansion, and acute clinical deterioration secondary to fourth ventricular or brainstem compression (see: Epidural Hematoma section) [133]. Venous sinus injury may alternatively lead to venous sinus thrombosis or dural AV fistula (See: Venous Injury section) [133].
The posterior skull base has several important foramina. The jugular foramen is at the posterior end of the petro-occipital fissure, extending laterally from the posterior occipital condyle. The sigmoid sinus and jugular bulb enter the jugular foramen at its posterior end. CN IX, X, XI enter the foramen at its anterior end. Damage to these cranial nerves is life-threatening. Deficits can include dysphagia, loss of the gag reflex, orthostatic hypotension, vocal cord paresis causing airway obstruction, upper GI dysmotility, and paralysis of sternocleidomastoid and trapezius muscles [133, 159].
The hypoglossal foramen carries CN XII, the meningeal branch of the ascending pharyngeal artery, and hypoglossal venous plexus. The hypoglossal foramen is adjacent to the occipital condyles. Fracture through this region is rare, and could result in ipsilateral tongue deviation and atrophy from CN XII palsy [133, 155].
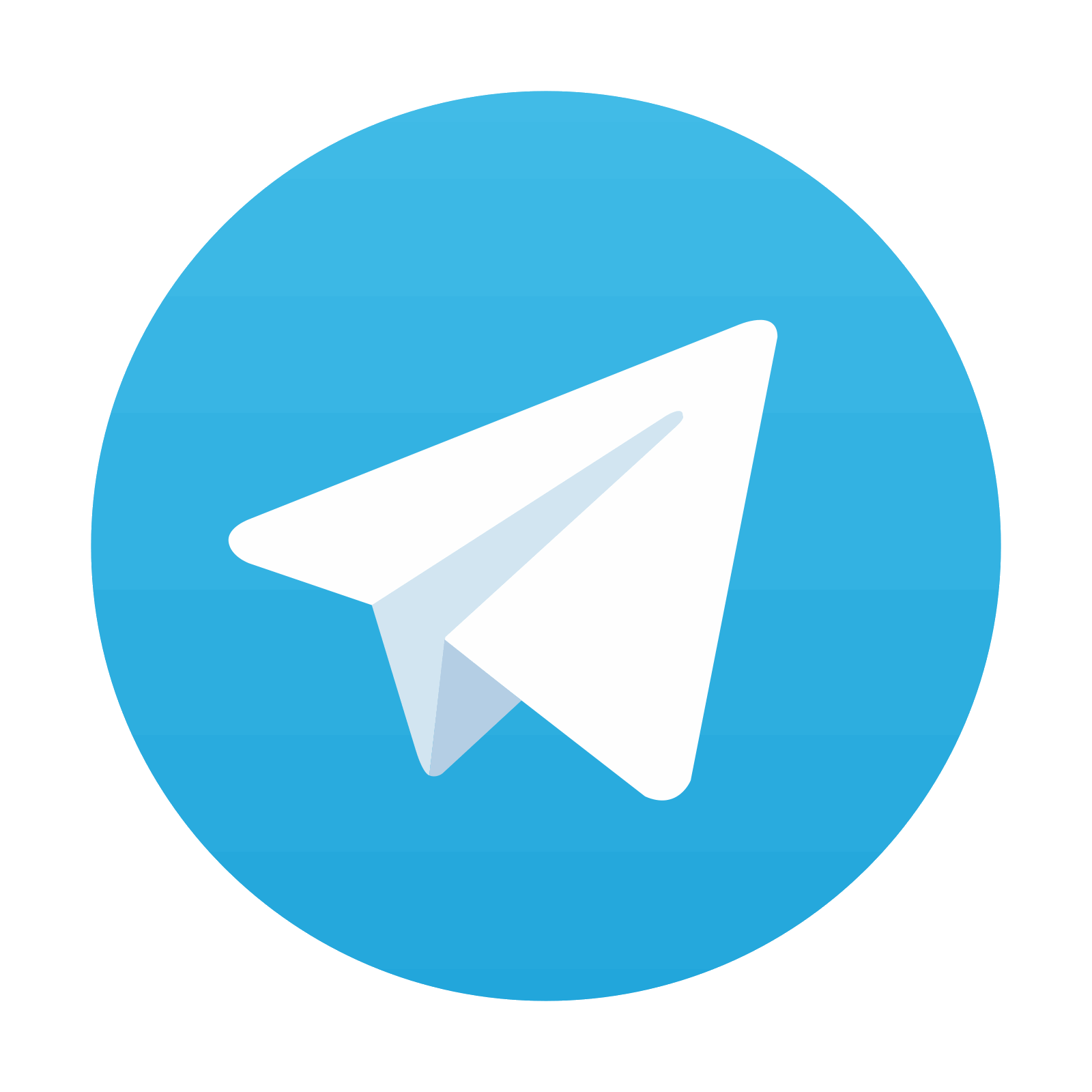
Stay updated, free articles. Join our Telegram channel
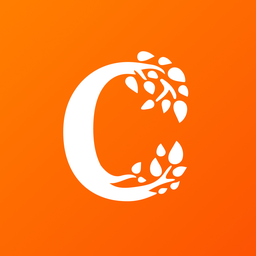
Full access? Get Clinical Tree
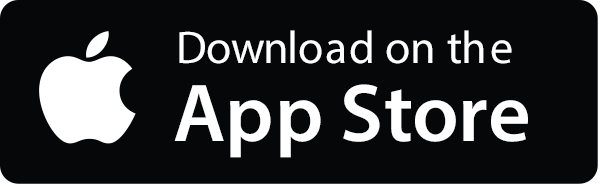
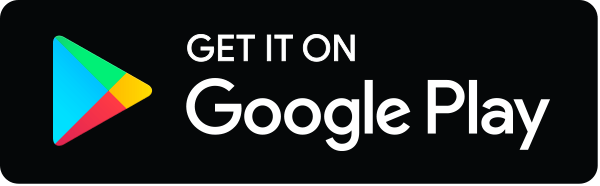