Eugene A. Lowry, MD; Stuart J. McKinnon, MD, PhD; and Yvonne Ou, MD
Neuroprotection is a therapeutic modality that aims to slow or prevent neuronal death. In glaucoma, the target neuron is the retinal ganglion cell (RGC) and its axon. The ultimate goal of neuroprotection is to delay or prevent the loss of visual function. An advantage of the neuroprotective approach is that regardless of the mechanism of glaucomatous optic nerve damage and visual loss, this strategy targets the final common pathway of neuronal loss at the level of the RGC and its axonal projections. Some argue that, by definition, glaucoma neuroprotection is independent from intraocular pressure (IOP) lowering.1 For the purposes of this discussion, we will follow this convention and define neuroprotection as therapy directed toward preserving RGC viability and function independent of IOP lowering.
PATHOGENESIS OF NEURONAL DEATH IN GLAUCOMA AND POSSIBLE THERAPEUTIC STRATEGIES
Although the exact mechanism of RGC death is unknown, several mechanisms have been proposed and studied. These include mechanical compression of RGC axons at the optic nerve head, decreased ocular perfusion pressure and ischemia, excitotoxicity, neurotrophic factor deprivation, oxidative stress, immune modulation, and exposures to molecules, such as nitric oxide, endothelin, and amyloid-β (Figure 75-1).2 The actual mechanism is likely to be multifactorial.
In direct axonal injury, 2 paradigms are most commonly discussed. One hypothesis is that axonal degeneration begins at the distal ends of the injured axon and spreads in a retrograde fashion toward the cell body.3 Alternatively, one or more focal injuries cause degeneration of the axon in both anterograde and retrograde directions, consistent with Wallerian degeneration. These mechanisms of axonal degeneration may not be mutually exclusive, as a severe local injury would be expected to have Wallerian degeneration, while a chronic focal injury may alter downstream metabolism with subsequent retrograde degeneration.4 It has been documented that axonal compression occurs as the axons pass through the lamina cribrosa of the optic nerve head, and it is hypothesized that this localized injury results in axonal transport disruption as well as glial activation, vascular ischemia, and hypoxia, all of which contribute to axonal degeneration.5,6
TARGETING APOPTOSIS
Despite the various proposed mechanisms of RGC death, the final common pathway likely involves apoptosis. This mode of programmed cell death has been seen in models of axotomy and optic nerve crush7–9 and in models of experimental glaucoma.10,11 It is thought that RGC death begins with damage at the lamina cribrosa, and therefore the RGC axon, and then spreads to the dendrites and soma in the retina.6,12 Murine crush models support this showing early phosphorylation of c-Jun N-terminal kinase (JNK) at the site of injury.13 JNKs have been shown to be involved in apoptosis through a variety of potential downstream mediators, including increased expression of Fas-L, BAK, and tumor necrosis factor alpha (TNF-α),14 and knockout mice for JNK show less RGC loss in crush models, arguing this may be one of the initiating apoptotic signals in glaucoma.13
Figure 75-1. Possible mechanisms leading to retinal ganglion cell apoptosis in glaucoma. Proposed mechanisms of retinal ganglion cell death include axon compression at the optic nerve head and blockage of axonal transport; decreased ocular perfusion pressure; excitotoxicity; activation of astrocytes, macrophages, and microglia; and exposure to molecules such as nitric oxide and amyloid-β. The role of cerebrospinal fluid pressure and its relationship to IOP is also under investigation. The actual mechanism is likely to include several different etiologies. (Reprinted with permission from Frankie-Lynn Silver, MD.)
Figure 75-2. The intrinsic and extrinsic apoptosis pathways. The extrinsic pathway is engaged by ligation of death receptors (eg, members of the TNFR superfamily), which results in procaspase-8 activation. Executioner caspases-3 and -7 are then activated, resulting in cell death. In the intrinsic pathway, a repertoire of BCL-2 proteins are activated, which results in mitochondrial outer membrane permeabilization (MOMP), cytochrome c release, and apoptosis inducing factor-1 (APAF-1)–dependent procaspase-9 activation. As with the extrinsic pathway, executioner caspases-3 and -7 are then activated. (Reprinted with permission from Macmillan Publishers Ltd. Chipuk JE, Green DR. Dissecting p53-dependent apoptosis. Cell Death and Differentiation. 2006;13:994-1002.)
Morphologically, the dying RGCs demonstrate dendrite and soma shrinkage, chromatin condensation, intracellular and DNA fragmentation, and formation of apoptotic bodies.12 Activation of a cascade of caspases, a class of cysteine proteases, culminates in apoptosis and cell death. Caspases are pro-proteins that become activated when the prodomain is cleaved off, and these activated caspases subsequently digest cellular contents. Caspases are activated by 2 distinct pathways: an extrinsic and an intrinsic pathway (Figure 75-2).15 In extrinsic apoptosis, also called the death receptor pathway, members of the TNF receptor superfamily are activated, leading to the formation of death-inducing signaling complexes with subsequent proteolytic cleavage of procaspase 8 and cell death.16 In intrinsic apoptosis, members of the BCL-2 gene family, principally Bax, are recruited to the mitochondrial outer membrane, resulting in loss of mitochondrial function and the release of cytochrome c, which complexes with a molecule called apoptosis-inducing factor-1 and procaspase 9. This complex causes proteolytic cleavage of procaspase 9, which activates the caspase cascade. Although these 2 pathways operate through different mechanisms, there appears to be overlap. Caspase 8 can activate the intrinsic pathway through activating a member of the BCL-2 family (BID) and Bax-deficient mice show reduced glia activation, which is thought to be a primary source of TNF-α.16 Interestingly, axonal degeneration may occur without somatic loss in Bax knockouts,17 while a murine crush model argues that axonal protection may be insufficient to preserve soma after axonal injury,18 arguing for the possibility of combined therapies protecting both axons and soma.
Strategies to target RGC apoptosis via these pathways include application or local expression of neurotrophic factors, which will be discussed in more detail below. It has been shown that neurotrophic factors can inhibit apoptosis by activating intracellular signaling pathways, such as the extracellular signal-regulated kinase 1/2 (Erk1/2), the phosphatidylinositol-3 kinase (PI3K), and the janus kinase/signal transducer and activator of transcription 3 (JAK/STAT3) pathways. The former 2 pathways activate the cAMP-response-element binding protein (CREB), which promotes transcription of several prosurvival genes, including BCL-2 and BCL-xL. Brain-derived neurotrophic factor (BDNF) promotes RGC survival via the Erk1/2 pathway, whereas ciliary-derived neurotrophic factor (CNTF) promotes RGC survival via the JAK/STAT3 pathway. Intravitreal injection of BDNF delays RGC death after axotomy and may be associated with the suppression of caspase 3 activity.19 Another strategy to target apoptosis is the use of caspase inhibitors. In rodent models of optic nerve axotomy and glaucoma, RGC apoptosis is reduced by intraocular administration of caspase inhibitors.20–23
TARGETING NEUROTROPHIC FACTORS
Neurotrophic factors promote neuronal survival and differentiation by activating survival signals and inhibiting apoptotic signaling cascades. It is hypothesized that blockage of axonal transport deprives RGCs from critical neurotrophic factors, resulting in RGC apoptosis. In the retina, the nerve growth factor family of neurotrophins includes BDNF, CNTF, nerve growth factor (NGF), neurotrophin-3 (NT3), and neurotrophin-4/5 (NT4/5).24 These neurotrophins bind to tyrosine receptor kinases (Trks) or the p75NT receptor (p75NTR) to promote either cell survival or cell death, respectively (Figure 75-3). BDNF is a potent neurotrophic factor for RGCs, and its retrograde transport is inhibited when IOP was acutely elevated in rats.25 Furthermore, the receptor for BDNF, TrkB, accumulated in the optic nerve head in the same model of experimental glaucoma.26 However, it should be noted that there are endogenous sources of neurotrophins in the retina, and they may compensate in situations when retrograde axonal transport of brain-derived neurotrophins is blocked.27–29 In a rat model of glaucoma, intravitreal injections of BDNF increased the number of surviving RGCs as compared to control eyes.30 In a similar model, viral vectors overexpressing BDNF were intravitreally injected in glaucomatous rat eyes and reduced RGC loss.31 Another study from the same group demonstrated that virally mediated overexpression of CNTF decreased RGC loss but not BDNF.32
Both NGF and CNTF have been studied as therapeutic compounds. Topical NGF has been shown to elevate levels in the retina and optic nerve of mouse models,33 and NGF has been applied topically in a variety of ocular diseases including optic gliomas, retinitis pigmentosa, and glaucoma with reasonable tolerability and early positive outcomes.34–36 In glaucoma, mouse models of glaucoma treated with NGF showed less RGC loss, and analysis in humans has shown possible promise but limited to case series in patients with end-stage glaucoma.34 CNTF, another trophic factor that is a member of the IL-6 cytokine family, has had more human research after earlier studies showing it promotes RGC survival and axonal regeneration in vitro and early animal models.37 An encapsulated cellular implant that secretes CNTF implant has been shown to maintain steady elevated CNTF levels more than 2 years after implantation.38 A phase I clinical trial (NCT01408472) investigated the outcomes of sustained release CNTF in patients with glaucoma, concluding in 2014 with no results released to date though was sufficiently promising that a phase II trial (NCT02862938) is underway.
There has been an association between brimonidine and increased levels of BDNF and other trophic factors in rodent models, although its mechanism of neuroprotection is incompletely understood.39,40 A multisite randomized study, the Low-Pressure Glacuoma Treatment Study (LoGTS), showed a significant decrease in visual field progression for patients treated with brimonidine 0.2% (9% progressed) compared to timolol 0.5% (39% progressed) despite equal IOP lowering. Patients in this study were examined, including with 24-2 HVF every 4 months, and determined to progress if a linear regression showed a negative slope confirmed at the next 2 consecutive appointments, requiring a minimum of 16 months assessment. Unfortunately, 36% of the brimonidine-treated group were lost to follow-up by 1 year (primarily due to ocular toxicity), though this was partially incorporated into the study design with an expectation of 20% loss to follow-up in the brimonidine group and there was no difference in IOP between those completing and not completing the first 16 months of follow-up in either treatment group. Still, the risk of attrition bias with differential follow-up and poorer tolerability of brimonidine have limited the impacts of this study.41
Figure 75-3. The balance of neurotrophin receptors and neurotrophin function. The actions of neurotrophins are mediated by 2 principal transmembrane-receptor signaling systems. Each neurotrophin receptor (TrkA, TrkB, TrkC, and p75NTR) is characterized by specific affinities for the neurotrophins (nerve growth factor [NGF], brain-derived neurotrophic factor [BDNF], neurotrophin-3 [NT3], and neurotrophin-4 [NT4]). Activation of the tyrosine receptor kinases (Trks) promotes cell survival, whereas activation of the p75NTR promotes cell death. (Reprinted with permission from Macmillan Publishers Ltd. Lu B, Pang PT, Woo NH. Nature Reviews Neuroscience. 2005;6:603-614.)
TARGETING GLUTAMATE EXCITOTOXICITY
Glutamate is one of the major excitatory neurotransmitters in the brain and visual system. Excessive neurotransmission initiated by glutamate can be excitotoxic and result in cell death, first discovered in the retina.42 There are 3 classes of ionotropic glutamate-gated ion channels: AMPA receptors, kainate receptors, and N-methyl-D-aspartate (NMDA) receptors,43 the last of which has been implicated in RGC loss. Under physiologic conditions, glutamate is released by presynaptic neurons, binds to post-synaptic receptors, and allows influx of cations and results in neurotransmission. However, overactivation of NMDA receptors due to excessive glutamate or receptor hyperactivity triggers excessive Ca2+ influx into neurons and initiates cell death pathways.43,44 High intracellular calcium results in mitochondrial membrane depolarization, release of cytochrome c into the cytosol, activation of caspases, and subsequent DNA fragmentation and apoptosis.
Despite the evidence that glutamate excitotoxicity results in RGC death, its role in glaucoma pathogenesis has been controversial. An early study45 found increased levels of glutamate in the vitreous of glaucoma vs control patients, but later studies were unable to confirm this finding.46–48 Certainly, it is also possible that injured or metabolically compromised RGCs may react to normal levels of glutamate abnormally, possibly through demonstrated differential receptor expression.43,49,50
Memantine is a derivative of the antiviral amantadine and is an NMDA channel blocker, and preferentially blocks its activity if the ion channel is excessively open (ie, in high glutamate conditions). When there is an increased concentration of extracellular glutamate, more NMDA receptors are activated and open, and memantine is more effective at blocking them under these conditions. Memantine has been shown to prevent or reduce neuronal death in numerous animal models of central nervous system injury, including stroke, Alzheimer’s disease (AD), and traumatic brain injury. In experimental primate models of glaucoma, memantine-inhibited RGC death and neuronal shrinkage in the lateral geniculate nucleus.51–54 However, primary endpoints were not reached in a human phase III clinical trial using memantine to treat primary open-angle glaucoma, detailed study results have not been released (NCT00141882 and NCT00168350), and to date Food and Drug Administration (FDA) approval for use in glaucoma has not been granted.
TARGETING AMYLOID-β
AD is the most common cause of dementia in older adults,55 characterized by progressive impairment in memory and cognitive function. As a neurodegenerative disease, major histologic features include neurofibrillary tangles and neuritic plaques. Amyloid precursor protein (APP) is a transmembrane protein expressed in the brain and RGCs and regulates neurite outgrowth, synaptogenesis, and cell survival. Cleavage of APP by γ- and β-secretases produces amyloid-β, which plays a major role in AD pathogenesis, while cleavage of APP β-secretase does not yield neurotoxic amyloid-β.
Several studies56–59 have raised the possibility that there may be a relationship between AD and glaucoma. Some studies have shown a higher incidence of glaucoma in patients with AD when compared to age-matched control patients and that patients with glaucoma are more likely to develop AD, though results in larger insurance-based data-sets have been conflicting.60–64 Structural studies of optic nerves from patients with AD have shown loss of RGCs.56,57 Increased amyloid-β may reduce axonal transport via RhoA or kinase-signaling pathways.65,66 Caspase activation and altered metabolism of APP and amyloid-β may contribute to RGC apoptosis in glaucoma.20,67
Currently, there are 2 classes of drugs approved to treat AD: acetylcholine esterase inhibitors (donepezil, rivastigmine, and galantamine) and an NMDA receptor antagonist (memantine). Other antiamyloid therapeutic strategies include decreasing amyloid-β production by inhibiting secretases, increasing the activity of β-secretases, or by interfering with amyloid-β aggregation using amyloid-β vaccination. In a rat glaucoma model, intravitreal injection of antibodies to amyloid-β or Congo red as well as β-secretase inhibitors decreased rates of RGC apoptosis.67
TARGETING NEUROINFLAMMATION
Recent evidence has focused attention on the possibility that inflammation plays a role in the pathogenesis of glaucoma. Early infiltration of the optic nerve by monocytes precedes glaucomatous damage in a mouse model, and inhibition of monocyte migration with a single radiation treatment can prevent glaucomatous nerve damage despite persistent elevation in IOP.68 Injured cells may express damage-associated molecular patterns (DAMPs), such as heat-shock proteins, which have been shown to be upregulated in patients with glaucoma compared with controls.69 These DAMPs activate astrocytes and microglia via toll-like receptors as well as the C1 complex of the complement cascade.70 It has been demonstrated that activation of the classical complement cascade results in RGC death. In the classic complement cascade, C1q binds to an antigen and activates several proteases (C1r, C1s, C2-C4) that initiate opsonization and attract phagocytes that directly attack cell membranes via the membrane attack complex (MAC). During development, C1q localizes to developing synapses and regulates synapse elimination.71 In the mature retina, activated microglia are a source of C1q and reduction of microglia activity with minocycline or genetic knockout of C1qa or C5 both improve RGC survival in murine models of glaucoma.72–75 In AD, damaged neuronal processes show evidence of MAC activation, with concurrent increased expression of C1q.76,77 C1q also binds to amyloid plaques in AD brains, reducing amyloid-β uptake and resulting in extracellular amyloid-β accumulation.78 C1q upregulation can be detected in the retinas of murine, primate, and human glaucomatous eyes.79 It was shown that complement activation in glaucoma resembles that seen in AD, raising the possibility that the normal developmental mechanism of complement-mediated synapse elimination becomes aberrantly reactivated in neurodegenerative diseases.71 However, the role of complement in glaucoma may not be purely destructive. In mouse experimental glaucoma models when axonal degeneration is inhibited by insertion of the Wld(S) gene, RGC degeneration is also inhibited, but there is still astrocyte activation and upregulation of C3. This is thought to be protective through EGFR signaling and knockouts of C3 or direct EGFR blockage had increased RGC death.80 Ultimately, a more tailored approach to complement regulation may therefore be required.
TARGETING TUMOR NECROSIS FACTOR SUPERFAMILY RECEPTOR PATHWAYS
TNF-α is a nonglycosylated protein cytokine with a molecular weight of 17 kDa and a length of 157 amino acids that can activate the extrinsic apoptosis cascade through signaling with its receptor TNFR1. TNF-α is produced by mast cells, neutrophils, activated lymphocytes, endothelial cells, adipocytes, and smooth muscle cells, as well as by natural killer cells after their stimulation by lipopolysaccharides. Prior studies have shown that that TNF-α and its membrane-bound receptors may contribute to the pathophysiology of glaucoma, although the mechanism of action is poorly understood. TNF-α production was upregulated in cultured rat retinal glial cells exposed to elevated hydrostatic pressure and caused apoptosis in cocultured rat RGCs.81 Immunohistochemical analysis has shown upregulation of TNF-α in the optic nerve head of human glaucoma patients, mainly in glia and ELISA assays of aqueous fluid in patients undergoing cataract surgeries showed higher expression of TNF-α in glaucoma patients vs controls.82 Using a laser mouse glaucoma model,83 Nakazawa and colleagues reported rapid upregulation of TNF-α, microglial activation, loss of optic nerve oligodendrocytes, and delayed loss of RGCs.84 This loss was reduced in mice with knockout for TNF or TNF-neutralizing antibodies as well as in vitro with RGC cells showing protection by the anti-TNF agent agmatine.84,85 Intravitreal TNF-α injections produced similar effects. Discordantly, murine models pretreated with intraocular TNF-α show less RGC loss after crush injury,86 and global TNF knockouts in ischemia models showed no change in rate of RGC loss.87 The differential responses to TNF-α may be partly dependent on the relative expression of competing receptors TNFR1 and TNFR2, with TNFR1 thought to signal cell death while TNFR2 may be neuroprotective. In both crush and ischemia models, TNFR1 knockouts showed neuroprotection.87,88 In contrast, TNFR2 knockouts showed enhanced rates of cell death, and TNFR2 activation leads to upregulation of a variety of anti-inflammatory genes.87–89
TARGETING DECREASED OCULAR PERFUSION AND HYPOXIA
As discussed in Chapter 73, decreased ocular perfusion pressure may play a role in glaucomatous optic neuropathy. Insufficient autoregulation of optic nerve blood flow may lead to optic nerve ischemia and RGC loss. In several animal studies,90,91 exogenous application of vasoactive peptide endothelin-1 resulting in decreased optic nerve blood flow can lead to RGC loss in the absence of elevated IOP, while bosentan (an endothelin receptor antagonist) has been shown to be protective.73
Hypoxia of the retina has also been shown to result in RGC apoptosis.92,93 The pathophysiology of neuronal hypoxic damage may involve several different mechanisms, including excitotoxic damage, calcium overload, and oxidative stress. Indeed, hypoxia-mediated mechanisms at work in the brain may play a role in glaucomatous optic neuropathy. There is evidence of vascular abnormalities in glaucoma patients, including vasospasm, systemic hypotension, and abnormal blood flow characteristics.94 There is also a clear association between retinal nerve fiber layer (RNFL) loss and loss of peripapillary vasculature on optical coherence tomography angiography, and greater circumpapillary capillary loss is associated with greater field defects.95,96 Recent comparisons of controls with preperimetric and perimetric normal-tension glaucoma found that while perimetric patients had both RNFL and capillary loss, pre-perimetric patients showed RNFL thinning without capillary dropout, suggesting capillary loss may be secondary.97 However, it is not entirely clear whether the loss of peripapillary vasculature is partially responsible for RNFL loss or a secondary effect of RNFL degeneration, with a lack of longitudinal studies with this relatively new technology. It was demonstrated that expression of hypoxia-inducible factor 1 alpha (HIF-1α) is upregulated in human glaucomatous retina and optic nerves compared to control patients.98 HIF-1α is an oxygen-regulated transcription factor that serves as a master regulator of oxygen homeostasis and is likely neuroprotective in the retina though a marker for relative ischemia.99
The most well-studied pharmacologic agent class that may improve perfusion of the optic nerve is calcium channel blockers that cause vasodilation of the cerebral vasculature. This class of medication has been evaluated in the context of normal-tension glaucoma treatment. Prospective clinical studies100–102 have shown that patients taking oral calcium channel blockers had fewer optic nerve and visual field changes as compared to control patients. However, concerns for systemic hypotension, especially nocturnal hypotension, have limited widespread use of this class of drugs.103
TARGETING OXIDATIVE STRESS
Oxidative stress reflects an imbalance between production of oxygen-free radicals and endogenous antioxidants. This can lead to cell death via necrotic or apoptotic pathways with activation of apoptosis signal-regulating kinase 1 (ASK1), a downstream mediator of the TNF-α signaling pathway.104 Patients with glaucoma have demonstrated lower levels of glutathione, an important antioxidant, and have higher levels of markers for oxidative stress, including nitric oxide and malondialdehyde.105–107 Antioxidants including tempol and α-lipoic acid have reduced RGC death in murine glaucoma models.108,109
TARGETING SCLERAL REMODELING
Extracellular matrix remodeling is observed in the course of glaucoma. In healthy controls, the collagen of the lamina cribrosa is smaller and more uniform than that of the sclera, but in patients with glaucoma, there is back-bowing of the lamina cribrosa with decreased overall collagen density and alteration of collagen subtypes with increased collage VI expression.110–112 Models of ocular biomechanics show that, in addition to IOP, scleral and lamina cribrosa stiffness are key predictors of biomechanical stress.113 Donor sclera of patients with glaucoma demonstrate increased peripapillary scleral stiffness compared with controls.114 It remains controversial whether the increased stiffness of glaucoma sclera represents a risk factor for progression or a compensatory protective mechanism. Mouse models of glaucoma treated with glyceraldehyde cross-linking to increase scleral stiffness had greater axonal loss than their untreated counterparts.115 Treatments with oral Losartan showed reduction in RGC loss in murine models associated with alterations in scleral biomechanics.116
TARGETING DUAL LEUCINE ZIPPER KINASE
Dual leucine zipper kinase (DLK) is a protein kinase that has been shown to phosphorylate MKK7, which itself is a known activator of JNK and associated apoptosis signaling pathways.117,118 High-throughput kinase screening with siRNA technology identified inhibition of DLK as neuroprotective in vitro for mouse RGCs.119 This finding was confirmed in vivo with both conditional knockout of DLK and treatment with a DLK inhibitor (tozasertib) reducing RGC loss in glaucoma models as well as in vitro with human RGCs using sunitinib.119,120 In rodent crush models, RGC soma loss is similarly reduced in DLK knockouts with reduced expression of JNK in the soma, though axonal regeneration was also reduced and JNK signaling preserved in the axons, raising the possibility that retained RGCs may not have preserved function.121,122
TARGETING METABOLIC VULNERABILITY
Given the high energy requirements for maintaining axonal resting potential and axonal transport of cellular products including trophic factors, cellular metabolism is increasingly recognized as a potential target for neuroprotection in glaucoma. RGCs, like other neurons, depend primarily on glucose for metabolism, which is taken up by high-affinity GLUT3 transporters as well as receiving significant metabolic support from surrounding glial cells.123 In DBA/2J mouse models of glaucoma, elevated IOP leads to decreased ATP levels and delayed recovery from oxygen-glucose deprivation in younger mice.124 Mitochondria of these mice are smaller with greater cristae disruption.125 In humans, patients with ocular hypertension had more efficient mitochondrial metabolism with higher conversion rates from ADP to ATP than either normal-tension glaucoma patients or controls. However, analysis was performed on peripheral lymphocytes, which may have metabolic differences from RGCs, and there were few differences between normal-tension patients and controls.126 Nicotinamide (vitamin B3) has recently emerged as a potential therapeutic candidate in glaucoma. Nicotinamide is a precursor to the metabolic intermediary NAD+ that is reduced to NADH during the citric acid cycle and supplies the electrons for oxidative metabolism. Cellular levels of NAD+ fall with aging.127,128 Among DBA/2J mice treated with the highest doses of nicotinamide, only 7% developed any evidence of optic neuropathy, compared with over 50% in controls.129
TARGETING HISTONE DEACETYLASE
Eukaryotic DNA is packaged in nucleosomes in which nucleic acid is wrapped around a core of histones.130 When nucleic acid and histones are in tight association, there is reduced access to DNA by transcription factors and, correspondingly, lower transcriptional activity.130 Acetylation of histone N-termini by histone acetlytransferases (HATs) reduces the positive charge of histones, decreasing their affinity for DNA and increasing transcriptional activity, while histone deacetylases (HDAC) have the opposite effect and reduce transcriptional activity.130 An imbalance in acetylation in either direction may lead to apoptosis and a relative decease in HAT, and corresponding hyper-deacetylation is an early finding in multiple neurodegenerative illnesses.131 In DBA/2J mice, reduced transcriptional activity and chromatin condensation has been found prior to Bax activation and RGC loss and is associated with increased HDAC activity.132–134 Valproic acid, which has myriad effects including inhibition of histone deacetylases, as well as the more specific HDAC inhibitor MS-275 and genetic knockouts of HDAC3, promote RGC survival in mouse models of optic nerve crush.135–137
TARGETING AXONAL REGENERATION
RGCs have minimal inherent regenerative potential after axonal injury in adult mammals. However, alteration in the cellular environment with peripheral nerve grafts or induction of inflammation enhances axonal regeneration in rats.138,139 Oncomodulin is released by macrophages and promotes axonal regrowth through calmodulin kinase-dependent signaling in a cAMP dependent manner both in vitro and in vivo in rat crush models.140 Regeneration potential is enhanced further by suppression of natural growth-inhibiting signals such as Nogo and PTEN, the latter of which activates the mTOR pathway.141–143 In models combining oncomodulin with PTEN deletion after transection, regenerating RGCs were able to extend to the LGN and superior colliculus.144 Further, these treated mice also had small but significant improvement in tracking compared with untreated controls that also had nerve transection, though both groups performed much worse than animals not undergoing nerve transection.144 Interestingly, this regenerative potential is also fostered by visual stimuli in combination with mTOR overexpression and the regenerating RGC subtypes appear to extend axons to appropriately selective visual centers though again functional visual improvements remained limited.145 RGCs may be divided by their morphology, genetic profile, and physiologic responses into as many as 30 subtypes.146 The specific subtype appears to make a difference in regenerative potential with several of the alpha-RGC subtypes having intrinsically higher mTOR expression and enhanced survival and regeneration after crush injury.147 Ultimately, in humans, regenerative therapies would not only require preservation of multiple subtypes of RGC soma and axon regrowth with myelination after the lamina cribrosa but also exquisite anatomic organization to maintain a retinotopic map for useful vision.
CONCLUSION
The field of neuroprotection in glaucoma is full of promise, but clinically proven strategies have remained elusive. While there has been accumulating laboratory evidence of the effectiveness of targeting RGC death as a treatment for glaucoma, data from randomized clinical trials demonstrating efficacy in humans are still lacking. One of the great challenges of neuroprotection clinical trials is that, compared with the fairly rapid response possible with IOP measurements, neuroprotective studies would be based on clinical or structural measurements such as nerve fiber layer measurements using optical coherence tomography and visual field testing. Given that these metrics may have greater variability (requiring larger populations) and take years to show a difference in effect (requiring extended follow-up), clinical trials of neuroprotective agents require significant financial investment compared with trials in which IOP lowering is the main outcome. Studies that focus on neuro-enhancement, acutely increasing the function of injured RGCs, may show improvements in functional metrics more rapidly.148 Additional recommendations to reduce these costs for neuroprotection include rapid in vitro screening, validation in multiple animal models before human testing, initial human testing with futility design to identify agents likely to have the greatest effect, and using registries to choose populations with less variability in subjective visual field testing.149 The search for successful neuroprotective therapies that act independently of IOP lowering is critically important and must continue to be an active area of research.
REFERENCES
1. Levin LA. Neuroprotection and regeneration in glaucoma. Ophthalmol Clin North Am. 2005;18(4):585-96, vii.
2. Kuehn MH, Fingert JH, Kwon YH. Retinal ganglion cell death in glaucoma: mechanisms and neuroprotective strategies. Ophthalmol Clin North Am. 2005;18(3):383-95, vi.
3. Cavanagh JB. The “dying back” process. A common denominator in many naturally occurring and toxic neuropathies. Arch Pathol Lab Med. 1979;103(13):659-664.
4. Nickells RW. The cell and molecular biology of glaucoma: mechanisms of retinal ganglion cell death. Invest Ophthalmol Vis Sci. 2012;53(5):2476-2481.
5. Quigley HA, Anderson DR. Distribution of axonal transport blockade by acute intraocular pressure elevation in the primate optic nerve head. Invest Ophthalmol Vis Sci. 1977;16(7):640-644.
6. Quigley HA, Addicks EM, Green WR, Maumenee AE. Optic nerve damage in human glaucoma. II. The site of injury and susceptibility to damage. Arch Ophthalmol. 1981;99(4):635-649.
7. Berkelaar M, Clarke DB, Wang YC, Bray GM, Aguayo AJ. Axotomy results in delayed death and apoptosis of retinal ganglion cells in adult rats. J Neurosci. 1994;14(7):4368-4374.
8. Garcia-Valenzuela E, Gorczyca W, Darzynkiewicz Z, Sharma SC. Apoptosis in adult retinal ganglion cells after axotomy. J Neurobiol. 1994;25(4):431-438.
9. Li Y, Schlamp CL, Nickells RW. Experimental induction of retinal ganglion cell death in adult mice. Invest Ophthalmol Vis Sci. 1999;40(5):1004-1008.
10. Quigley HA, Nickells RW, Kerrigan LA, Pease ME, Thibault DJ, Zack DJ. Retinal ganglion cell death in experimental glaucoma and after axotomy occurs by apoptosis. Invest Ophthalmol Vis Sci. 1995;36(5):774-786.
11. Garcia-Valenzuela E, Shareef S, Walsh J, Sharma SC. Programmed cell death of retinal ganglion cells during experimental glaucoma. Exp Eye Res. 1995;61(1):33-44.
12. Howell GR, Libby RT, Jakobs TC, et al. Axons of retinal ganglion cells are insulted in the optic nerve early in DBA/2J glaucoma. J Cell Biol. 2007;179(7):1523-1537.
13. Fernandes KA, Harder JM, Fornarola LB, et al. JNK2 and JNK3 are major regulators of axonal injury-induced retinal ganglion cell death. Neurobiol Dis. 2012;46(2):393-401.
14. Dhanasekaran DN, Reddy EP. JNK signaling in apoptosis. Oncogene. 2008;27(48):6245-6251.
15. Nickells RW, Semaan SJ, Schlamp CL. Involvement of the Bcl2 gene family in the signaling and control of retinal ganglion cell death. Prog Brain Res. 2008;173:423-435.
16. Maes ME, Schlamp CL, Nickells RW. BAX to basics: how the BCL2 gene family controls the death of retinal ganglion cells. Prog Retin Eye Res. 2017;57:1-25.
17. Libby RT, Li Y, Savinova OV, et al. Susceptibility to neurodegeneration in a glaucoma is modified by bax gene dosage. PLoS Genet. 2005;1(1):17-26.
18. Beirowski B, Babetto E, Coleman MP, Martin KR. The WldS gene delays axonal but not somatic degeneration in a rat glaucoma model. Eur J Neurosci. 2008;28(6):1166-1179.
19. Klocker N, Kermer P, Weishaupt JH, Labes M, Ankerhold R, Bahr M. Brain-derived neurotrophic factor-mediated neuroprotection of adult rat retinal ganglion cells in vivo does not exclusively depend on phosphatidyl-inositol-3’-kinase/protein kinase B signaling. J Neurosci. 2000;20(18):6962-6967.
20. McKinnon SJ, Lehman DM, Kerrigan-Baumrind LA, et al. Caspase activation and amyloid precursor protein cleavage in rat ocular hypertension. Invest Ophthalmol Vis Sci. 2002;43(4):1077-1087.
21. Hanninen VA, Pantcheva MB, Freeman EE, Poulin NR, Grosskreutz CL. Activation of caspase 9 in a rat model of experimental glaucoma. Curr Eye Res. 2002;25(6):389-395.
22. Chaudhary P, Ahmed F, Quebada P, Sharma SC. Caspase inhibitors block the retinal ganglion cell death following optic nerve transection. Brain Res Mol Brain Res. 1999;67(1):36-45.
23. Kermer P, Ankerhold R, Klocker N, Krajewski S, Reed JC, Bahr M. Caspase-9: involvement in secondary death of axotomized rat retinal ganglion cells in vivo. Brain Res Mol Brain Res. 2000;85(1-2):144-150.
24. Johnson EC, Guo Y, Cepurna WO, Morrison JC. Neurotrophin roles in retinal ganglion cell survival: lessons from rat glaucoma models. Exp Eye Res. 2009;88(4):808-815.
25. Quigley HA, McKinnon SJ, Zack DJ, et al. Retrograde axonal transport of BDNF in retinal ganglion cells is blocked by acute IOP elevation in rats. Invest Ophthalmol Vis Sci. 2000;41(11):3460-3466.
26. Pease ME, McKinnon SJ, Quigley HA, Kerrigan-Baumrind LA, Zack DJ. Obstructed axonal transport of BDNF and its receptor TrkB in experimental glaucoma. Invest Ophthalmol Vis Sci. 2000;41(3):764-774.
27. Vecino E, Garcia-Crespo D, Garcia M, Martinez-Millan L, Sharma SC, Carrascal E. Rat retinal ganglion cells co-express brain derived neurotrophic factor (BDNF) and its receptor TrkB. Vision Res. 2002;42(2):151-157.
28. Spalding KL, Rush RA, Harvey AR. Target-derived and locally derived neurotrophins support retinal ganglion cell survival in the neonatal rat retina. J Neurobiol. 2004;60(3):319-327.
29. Seki M, Tanaka T, Sakai Y, et al. Muller cells as a source of brain-derived neurotrophic factor in the retina: noradrenaline upregulates brain-derived neurotrophic factor levels in cultured rat muller cells. Neurochem Res. 2005;30(9):1163-1170.
30. Kalback W, Watson MD, Kokjohn TA, et al. APP transgenic mice Tg2576 accumulate abeta peptides that are distinct from the chemically modified and insoluble peptides deposited in Alzheimer’s disease senile plaques. Biochemistry. 2002;41(3):922-928.
31. Martin KR, Quigley HA, Zack DJ, et al. Gene therapy with brain-derived neurotrophic factor as a protection: retinal ganglion cells in a rat glaucoma model. Invest Ophthalmol Vis Sci. 2003;44(10):4357-4365.
32. Pease ME, Zack DJ, Berlinicke C, et al. Effect of CNTF on retinal ganglion cell survival in experimental glaucoma. Invest Ophthalmol Vis Sci. 2009;50(5):2194-2200.
33. Lambiase A, Tirassa P, Micera A, Aloe L, Bonini S. Pharmacokinetics of conjunctivally applied nerve growth factor in the retina and optic nerve of adult rats. Invest Ophthalmol Vis Sci. 2005;46(10):3800-3806.
34. Lambiase A, Aloe L, Centofanti M, et al. Experimental and clinical evidence of neuroprotection by nerve growth factor eye drops: implications for glaucoma. Proc Natl Acad Sci U S A. 2009;106(32):13469-13474.
35. Falsini B, Chiaretti A, Rizzo D, et al. Nerve growth factor improves visual loss in childhood optic gliomas: a randomized, double-blind, phase II clinical trial. Brain. 2016;139(pt 2):404-414.
36. Falsini B, Iarossi G, Chiaretti A, et al. NGF eye-drops topical administration in patients with retinitis pigmentosa, a pilot study. J Transl Med. 2016;14:8-015-0750-3.
37. Kimura A, Namekata K, Guo X, Harada C, Harada T. Neuroprotection, growth factors and BDNF-TrkB signalling in retinal degeneration. Int J Mol Sci. 2016;17(9):E1584.
38. Kauper K, McGovern C, Sherman S, et al. Two-year intraocular delivery of ciliary neurotrophic factor by encapsulated cell technology implants in patients with chronic retinal degenerative diseases. Invest Ophthalmol Vis Sci. 2012;53(12):7484-7491.
39. Gao H, Qiao X, Cantor LB, WuDunn D. Up-regulation of brain-derived neurotrophic factor expression by brimonidine in rat retinal ganglion cells. Arch Ophthalmol. 2002;120(6):797-803.
40. Lonngren U, Napankangas U, Lafuente M, et al. The growth factor response in ischemic rat retina and superior colliculus after brimonidine pre-treatment. Brain Res Bull. 2006;71(1-3):208-218.
41. Krupin T, Liebmann JM, Greenfield DS, Ritch R, Gardiner S, Low-Pressure Glaucoma Study Group. A randomized trial of brimonidine versus timolol in preserving visual function: results from the low-pressure glaucoma treatment study. Am J Ophthalmol. 2011;151(4):671-681.
42. Lucas DR, Newhouse JP. The toxic effect of sodium L-glutamate on the inner layers of the retina. AMA Arch Ophthalmol. 1957;58(2):193-201.
43. Seki M, Lipton SA. Targeting excitotoxic/free radical signaling pathways for therapeutic intervention in glaucoma. Prog Brain Res. 2008;173:495-510.
44. Lipton SA, Rosenberg PA. Excitatory amino acids as a final common pathway for neurologic disorders. N Engl J Med. 1994;330(9):613-622.
45. Dreyer EB, Zurakowski D, Schumer RA, Podos SM, Lipton SA. Elevated glutamate levels in the vitreous body of humans and monkeys with glaucoma. Arch Ophthalmol. 1996;114(3):299-305.
46. Honkanen RA, Baruah S, Zimmerman MB, et al. Vitreous amino acid concentrations in patients with glaucoma undergoing vitrectomy. Arch Ophthalmol. 2003;121(2):183-188.
47. Levkovitch-Verbin H, Martin KR, Quigley HA, Baumrind LA, Pease ME, Valenta D. Measurement of amino acid levels in the vitreous humor of rats after chronic intraocular pressure elevation or optic nerve transection. J Glaucoma. 2002;11(5):396-405.
48. Wamsley S, Gabelt BT, Dahl DB, et al. Vitreous glutamate concentration and axon loss in monkeys with experimental glaucoma. Arch Ophthalmol. 2005;123(1):64-70.
49. Naskar R, Vorwerk CK, Dreyer EB. Concurrent downregulation of a glutamate transporter and receptor in glaucoma. Invest Ophthalmol Vis Sci. 2000;41(7):1940-1944.
50. Ju WK, Kim KY, Noh YH, et al. Increased mitochondrial fission and volume density by blocking glutamate excitotoxicity protect glaucomatous optic nerve head astrocytes. Glia. 2015;63(5):736-753.
51. WoldeMussie E, Yoles E, Schwartz M, Ruiz G, Wheeler LA. Neuroprotective effect of memantine in different retinal injury models in rats. J Glaucoma. 2002;11(6):474-480.
52. Yucel YH, Gupta N, Zhang Q, Mizisin AP, Kalichman MW, Weinreb RN. Memantine protects neurons from shrinkage in the lateral geniculate nucleus in experimental glaucoma. Arch Ophthalmol. 2006;124(2):217-225.
53. Hare WA, WoldeMussie E, Lai RK, et al. Efficacy and safety of memantine treatment for reduction of changes associated with experimental glaucoma in monkey, I: functional measures. Invest Ophthalmol Vis Sci. 2004;45(8):2625-2639.
54. Hare WA, WoldeMussie E, Weinreb RN, et al. Efficacy and safety of memantine treatment for reduction of changes associated with experimental glaucoma in monkey, II: structural measures. Invest Ophthalmol Vis Sci. 2004;45(8):2640-2651.
55. Reitz C, Brayne C, Mayeux R. Epidemiology of Alzheimer disease. Nat Rev Neurol. 2011;7(3):137-152.
56. Hinton DR, Sadun AA, Blanks JC, Miller CA. Optic-nerve degeneration in Alzheimer’s disease. N Engl J Med. 1986;315(8):485-487.
57. Sadun AA, Bassi CJ. Optic nerve damage in Alzheimer’s disease. Ophthalmology. 1990;97(1):9-17.
58. Bayer AU, Keller ON, Ferrari F, Maag KP. Association of glaucoma with neurodegenerative diseases with apoptotic cell death: Alzheimer’s disease and Parkinson’s disease. Am J Ophthalmol. 2002;133(1):135-137.
59. Tamura H, Kawakami H, Kanamoto T, et al. High frequency of open-angle glaucoma in japanese patients with Alzheimer’s disease. J Neurol Sci. 2006;246(1-2):79-83.
60. Helmer C, Malet F, Rougier MB, et al. Is there a link between open-angle glaucoma and dementia? The three-city-alienor cohort. Ann Neurol. 2013;74(2):171-179.
61. Ou Y, Grossman DS, Lee PP, Sloan FA. Glaucoma, Alzheimer disease and other dementia: a longitudinal analysis. Ophthalmic Epidemiol. 2012;19(5):285-292.
62. Chung SD, Ho JD, Chen CH, Lin HC, Tsai MC, Sheu JJ. Dementia is associated with open-angle glaucoma: a population-based study. Eye (Lond). 2015;29(10):1340-1346.
63. Lin IC, Wang YH, Wang TJ, et al. Glaucoma, Alzheimer’s disease, and Parkinson’s disease: an 8-year population-based follow-up study. PLoS One. 2014;9(9):e108938.
64. Bayer AU, Ferrari F, Erb C. High occurrence rate of glaucoma among patients with Alzheimer’s disease. Eur Neurol. 2002;47(3):165-168.
65. Decker H, Lo KY, Unger SM, Ferreira ST, Silverman MA. Amyloid-beta peptide oligomers disrupt axonal transport through an NMDA receptor-dependent mechanism that is mediated by glycogen synthase kinase 3beta in primary cultured hippocampal neurons. J Neurosci. 2010;30(27):9166-9171.
66. Tsushima H, Emanuele M, Polenghi A, et al. HDAC6 and RhoA are novel players in abeta-driven disruption of neuronal polarity. Nat Commun. 2015;6:7781.
67. Guo L, Salt TE, Luong V, et al. Targeting amyloid-beta in glaucoma treatment. Proc Natl Acad Sci U S A. 2007;104(33):13444-13449.
68. Howell GR, Soto I, Zhu X, et al. Radiation treatment inhibits monocyte entry into the optic nerve head and prevents neuronal damage in a mouse model of glaucoma. J Clin Invest. 2012;122(4):1246-1261.
69. Tezel G, Hernandez R, Wax MB. Immunostaining of heat shock proteins in the retina and optic nerve head of normal and glaucomatous eyes. Arch Ophthalmol. 2000;118(4):511-518.
70. Yu L, Wang L, Chen S. Endogenous toll-like receptor ligands and their biological significance. J Cell Mol Med. 2010;14(11):2592-2603.
71. Stevens B, Allen NJ, Vazquez LE, et al. The classical complement cascade mediates CNS synapse elimination. Cell. 2007;131(6):1164-1178.
72. Howell GR, Soto I, Ryan M, Graham LC, Smith RS, John SW. Deficiency of complement component 5 ameliorates glaucoma in DBA/2J mice. J Neuroinflammation. 2013;10:76-2094-10-76.
73. Howell GR, Macalinao DG, Sousa GL, et al. Molecular clustering identifies complement and endothelin induction as early events in a mouse model of glaucoma. J Clin Invest. 2011;121(4):1429-1444.
74. Bosco A, Inman DM, Steele MR, et al. Reduced retina microglial activation and improved optic nerve integrity with minocycline treatment in the DBA/2J mouse model of glaucoma. Invest Ophthalmol Vis Sci. 2008;49(4):1437-1446.
75. Williams PA, Tribble JR, Pepper KW, et al. Inhibition of the classical pathway of the complement cascade prevents early dendritic and synaptic degeneration in glaucoma. Mol Neurodegener. 2016;11:26-016-0091-6.
76. Itagaki S, Akiyama H, Saito H, McGeer PL. Ultrastructural localization of complement membrane attack complex (MAC)-like immunoreactivity in brains of patients with Alzheimer’s disease. Brain Res. 1994;645(1-2):78-84.
77. Emmerling MR, Watson MD, Raby CA, Spiegel K. The role of complement in Alzheimer’s disease pathology. Biochim Biophys Acta. 2000;1502(1):158-171.
78. Webster SD, Yang AJ, Margol L, Garzon-Rodriguez W, Glabe CG, Tenner AJ. Complement component C1q modulates the phagocytosis of abeta by microglia. Exp Neurol. 2000;161(1):127-138.
79. Stasi K, Nagel D, Yang X, et al. Complement component 1Q (C1Q) upregulation in retina of murine, primate, and human glaucomatous eyes. Invest Ophthalmol Vis Sci. 2006;47(3):1024-1029.
80. Harder JM, Braine CE, Williams PA, et al. Early immune responses are independent of RGC dysfunction in glaucoma with complement component C3 being protective. Proc Natl Acad Sci U S A. 2017;114(19):E3839-E3848.
81. Tezel G, Wax MB. Increased production of tumor necrosis factor-alpha by glial cells exposed to simulated ischemia or elevated hydrostatic pressure induces apoptosis in cocultured retinal ganglion cells. J Neurosci. 2000;20(23):8693-8700.
82. Sawada H, Fukuchi T, Tanaka T, Abe H. Tumor necrosis factor-alpha concentrations in the aqueous humor of patients with glaucoma. Invest Ophthalmol Vis Sci. 2010;51(2):903-906.
83. Tezel G, Li LY, Patil RV, Wax MB. TNF-alpha and TNF-alpha receptor-1 in the retina of normal and glaucomatous eyes. Invest Ophthalmol Vis Sci. 2001;42(8):1787-1794.
84. Nakazawa T, Nakazawa C, Matsubara A, et al. Tumor necrosis factor-alpha mediates oligodendrocyte death and delayed retinal ganglion cell loss in a mouse model of glaucoma. J Neurosci. 2006;26(49):12633-12641.
85. Hong S, Kim CY, Lee JE, Seong GJ. Agmatine protects cultured retinal ganglion cells from tumor necrosis factor-alpha-induced apoptosis. Life Sci. 2009;84(1-2):28-32.
86. Mac Nair CE, Fernandes KA, Schlamp CL, Libby RT, Nickells RW. Tumor necrosis factor alpha has an early protective effect on retinal ganglion cells after optic nerve crush. J Neuroinflammation. 2014;11:194-014-0194-3.
87. Fontaine V, Mohand-Said S, Hanoteau N, Fuchs C, Pfizenmaier K, Eisel U. Neurodegenerative and neuroprotective effects of tumor necrosis factor (TNF) in retinal ischemia: opposite roles of TNF receptor 1 and TNF receptor 2. J Neurosci. 2002;22(7):RC216.
88. Tezel G, Yang X, Yang J, Wax MB. Role of tumor necrosis factor receptor-1 in the death of retinal ganglion cells following optic nerve crush injury in mice. Brain Res. 2004;996(2):202-212.
89. Veroni C, Gabriele L, Canini I, et al. Activation of TNF receptor 2 in microglia promotes induction of anti-inflammatory pathways. Mol Cell Neurosci. 2010;45(3):234-244.
90. Orgul S, Cioffi GA, Bacon DR, Van Buskirk EM. An endothelin-1-induced model of chronic optic nerve ischemia in rhesus monkeys. J Glaucoma. 1996;5(2):135-138.
91. Chauhan BC, LeVatte TL, Jollimore CA, et al. Model of endothelin-1-induced chronic optic neuropathy in rat. Invest Ophthalmol Vis Sci. 2004;45(1):144-152.
92. Kitano S, Morgan J, Caprioli J. Hypoxic and excitotoxic damage to cultured rat retinal ganglion cells. Exp Eye Res. 1996;63(1):105-112.
93. Gross RL, Hensley SH, Gao F, Wu SM. Retinal ganglion cell dysfunction induced by hypoxia and glutamate: potential neuroprotective effects of beta-blockers. Surv Ophthalmol. 1999;43(suppl 1):S162-S170.
94. Flammer J, Orgul S, Costa VP, et al. The impact of ocular blood flow in glaucoma. Prog Retin Eye Res. 2002;21(4):359-393.
95. Yarmohammadi A, Zangwill LM, Diniz-Filho A, et al. Relationship between optical coherence tomography angiography vessel density and severity of visual field loss in glaucoma. Ophthalmology. 2016;123(12):2498-2508.
96. Lee EJ, Kim S, Hwang S, Han JC, Kee C. Microvascular compromise develops following nerve fiber layer damage in normal-tension glaucoma without choroidal vasculature involvement. J Glaucoma. 2017;26(3):216-222.
97. Kim SB, Lee EJ, Han JC, Kee C. Comparison of peripapillary vessel density between preperimetric and perimetric glaucoma evaluated by OCT-angiography. PLoS One. 2017;12(8):e0184297.
98. Tezel G, Wax MB. Hypoxia-inducible factor 1alpha in the glaucomatous retina and optic nerve head. Arch Ophthalmol. 2004;122(9):1348-1356.
99. Cheng L, Yu H, Yan N, Lai K, Xiang M. Hypoxia-inducible factor-1alpha target genes contribute to retinal neuroprotection. Front Cell Neurosci. 2017;11:20.
100. Netland PA, Chaturvedi N, Dreyer EB. Calcium channel blockers in the management of low-tension and open-angle glaucoma. Am J Ophthalmol. 1993;115(5):608-613.
101. Sawada A, Kitazawa Y, Yamamoto T, Okabe I, Ichien K. Prevention of visual field defect progression with brovincamine in eyes with normal-tension glaucoma. Ophthalmology. 1996;103(2):283-288.
102. Bose S, Piltz JR, Breton ME. Nimodipine, a centrally active calcium antagonist, exerts a beneficial effect on contrast sensitivity in patients with normal-tension glaucoma and in control subjects. Ophthalmology. 1995;102(8):1236-1241.
103. Hayreh SS, Zimmerman MB, Podhajsky P, Alward WL. Nocturnal arterial hypotension and its role in optic nerve head and ocular ischemic disorders. Am J Ophthalmol. 1994;117(5):603-624.
104. Kimura A, Namekata K, Guo X, Noro T, Harada C, Harada T. Targeting oxidative stress for treatment of glaucoma and optic neuritis. Oxid Med Cell Longev. 2017;2017:2817252.
105. Gherghel D, Griffiths HR, Hilton EJ, Cunliffe IA, Hosking SL. Systemic reduction in glutathione levels occurs in patients with primary open-angle glaucoma. Invest Ophthalmol Vis Sci. 2005;46(3):877-883.
106. Gherghel D, Mroczkowska S, Qin L. Reduction in blood glutathione levels occurs similarly in patients with primary-open angle or normal tension glaucoma. Invest Ophthalmol Vis Sci. 2013;54(5):3333-3339.
107. Benoist d’Azy C, Pereira B, Chiambaretta F, Dutheil F. Oxidative and anti-oxidative stress markers in chronic glaucoma: a systematic review and meta-analysis. PLoS One. 2016;11(12):e0166915.
108. Inman DM, Lambert WS, Calkins DJ, Horner PJ. Alpha-lipoic acid antioxidant treatment limits glaucoma-related retinal ganglion cell death and dysfunction. PLoS One. 2013;8(6):e65389.
109. Yang X, Hondur G, Tezel G. Antioxidant treatment limits neuroinflammation in experimental glaucoma. Invest Ophthalmol Vis Sci. 2016;57(4):2344-2354.
110. Quigley HA, Hohman RM, Addicks EM, Massof RW, Green WR. Morphologic changes in the lamina cribrosa correlated with neural loss in open-angle glaucoma. Am J Ophthalmol. 1983;95(5):673-691.
111. Quigley HA, Dorman-Pease ME, Brown AE. Quantitative study of collagen and elastin of the optic nerve head and sclera in human and experimental monkey glaucoma. Curr Eye Res. 1991;10(9):877-888.
112. Hernandez MR, Andrzejewska WM, Neufeld AH. Changes in the extracellular matrix of the human optic nerve head in primary open-angle glaucoma. Am J Ophthalmol. 1990;109(2):180-188.
113. Sigal IA, Flanagan JG, Ethier CR. Factors influencing optic nerve head biomechanics. Invest Ophthalmol Vis Sci. 2005;46(11):4189-4199.
114. Coudrillier B, Tian J, Alexander S, Myers KM, Quigley HA, Nguyen TD. Biomechanics of the human posterior sclera: age- and glaucoma-related changes measured using inflation testing. Invest Ophthalmol Vis Sci. 2012;53(4):1714-1728.
115. Kimball EC, Nguyen C, Steinhart MR, et al. Experimental scleral cross-linking increases glaucoma damage in a mouse model. Exp Eye Res. 2014;128:129-140.
116. Quigley HA, Pitha IF, Welsbie DS, et al. Losartan treatment protects retinal ganglion cells and alters scleral remodeling in experimental glaucoma. PLoS One. 2015;10(10):e0141137.
117. Merritt SE, Mata M, Nihalani D, Zhu C, Hu X, Holzman LB. The mixed lineage kinase DLK utilizes MKK7 and not MKK4 as substrate. J Biol Chem. 1999;274(15):10195-10202.
118. Tournier C, Whitmarsh AJ, Cavanagh J, Barrett T, Davis RJ. Mitogen-activated protein kinase kinase 7 is an activator of the c-jun NH2-terminal kinase. Proc Natl Acad Sci U S A. 1997;94(14):7337-7342.
119. Welsbie DS, Yang Z, Ge Y, et al. Functional genomic screening identifies dual leucine zipper kinase as a key mediator of retinal ganglion cell death. Proc Natl Acad Sci U S A. 2013;110(10):4045-4050.
120. Welsbie DS, Mitchell KL, Jaskula-Ranga V, et al. Enhanced functional genomic screening identifies novel mediators of dual leucine zipper kinase-dependent injury signaling in neurons. Neuron. 2017;94(6):1142-1154.e6.
121. Watkins TA, Wang B, Huntwork-Rodriguez S, et al. DLK initiates a transcriptional program that couples apoptotic and regenerative responses to axonal injury. Proc Natl Acad Sci U S A. 2013;110(10):4039-4044.
122. Fernandes KA, Harder JM, John SW, Shrager P, Libby RT. DLK-dependent signaling is important for somal but not axonal degeneration of retinal ganglion cells following axonal injury. Neurobiol Dis. 2014;69:108-116.
123. Inman DM, Harun-Or-Rashid M. Metabolic vulnerability in the neurodegenerative disease glaucoma. Front Neurosci. 2017;11:146.
124. Baltan S, Inman DM, Danilov CA, Morrison RS, Calkins DJ, Horner PJ. Metabolic vulnerability disposes retinal ganglion cell axons to dysfunction in a model of glaucomatous degeneration. J Neurosci. 2010;30(16):5644-5652.
125. Coughlin L, Morrison RS, Horner PJ, Inman DM. Mitochondrial morphology differences and mitophagy deficit in murine glaucomatous optic nerve. Invest Ophthalmol Vis Sci. 2015;56(3):1437-1446.
126. Lascaratos G, Chau KY, Zhu H, et al. Resistance to the most common optic neuropathy is associated with systemic mitochondrial efficiency. Neurobiol Dis. 2015;82:78-85.
127. Mouchiroud L, Houtkooper RH, Moullan N, et al. The NAD(+)/sirtuin pathway modulates longevity through activation of mitochondrial UPR and FOXO signaling. Cell. 2013;154(2):430-441.
128. Ramsey KM, Mills KF, Satoh A, Imai S. Age-associated loss of Sirt1-mediated enhancement of glucose-stimulated insulin secretion in beta cell-specific Sirt1-overexpressing (BESTO) mice. Aging Cell. 2008;7(1):78-88.
129. Williams PA, Harder JM, Foxworth NE, et al. Vitamin B3 modulates mitochondrial vulnerability and prevents glaucoma in aged mice. Science. 2017;355(6326):756-760.
130. Grunstein M. Histone acetylation in chromatin structure and transcription. Nature. 1997;389(6649):349-352.
131. Saha RN, Pahan K. HATs and HDACs in neurodegeneration: a tale of disconcerted acetylation homeostasis. Cell Death Differ. 2006;13(4):539-550.
132. Soto I, Oglesby E, Buckingham BP, et al. Retinal ganglion cells down-regulate gene expression and lose their axons within the optic nerve head in a mouse glaucoma model. J Neurosci. 2008;28(2):548-561.
133. Pelzel HR, Schlamp CL, Waclawski M, Shaw MK, Nickells RW. Silencing of Fem1cR3 gene expression in the DBA/2J mouse precedes retinal ganglion cell death and is associated with histone deacetylase activity. Invest Ophthalmol Vis Sci. 2012;53(3):1428-1435.
134. Schmitt HM, Schlamp CL, Nickells RW. Role of HDACs in optic nerve damage-induced nuclear atrophy of retinal ganglion cells. Neurosci Lett. 2016;625:11-15.
135. Biermann J, Grieshaber P, Goebel U, et al. Valproic acid-mediated neuroprotection and regeneration in injured retinal ganglion cells. Invest Ophthalmol Vis Sci. 2010;51(1):526-534.
136. Chindasub P, Lindsey JD, Duong-Polk K, Leung CK, Weinreb RN. Inhibition of histone deacetylases 1 and 3 protects injured retinal ganglion cells. Invest Ophthalmol Vis Sci. 2013;54(1):96-102.
137. Schmitt HM, Pelzel HR, Schlamp CL, Nickells RW. Histone deacetylase 3 (HDAC3) plays an important role in retinal ganglion cell death after acute optic nerve injury. Mol Neurodegener. 2014;9:39-1326-9-39.
138. Berry M, Carlile J, Hunter A. Peripheral nerve explants grafted into the vitreous body of the eye promote the regeneration of retinal ganglion cell axons severed in the optic nerve. J Neurocytol. 1996;25(2):147-170.
139. Leon S, Yin Y, Nguyen J, Irwin N, Benowitz LI. Lens injury stimulates axon regeneration in the mature rat optic nerve. J Neurosci. 2000;20(12):4615-4626.
140. Yin Y, Henzl MT, Lorber B, et al. Oncomodulin is a macrophage-derived signal for axon regeneration in retinal ganglion cells. Nat Neurosci. 2006;9(6):843-852.
141. Fischer D, He Z, Benowitz LI. Counteracting the nogo receptor enhances optic nerve regeneration if retinal ganglion cells are in an active growth state. J Neurosci. 2004;24(7):1646-1651.
142. Kurimoto T, Yin Y, Omura K, et al. Long-distance axon regeneration in the mature optic nerve: contributions of oncomodulin, cAMP, and pten gene deletion. J Neurosci. 2010;30(46):15654-15663.
143. Park KK, Liu K, Hu Y, et al. Promoting axon regeneration in the adult CNS by modulation of the PTEN/mTOR pathway. Science. 2008;322(5903):963-966.
144. de Lima S, Koriyama Y, Kurimoto T, et al. Full-length axon regeneration in the adult mouse optic nerve and partial recovery of simple visual behaviors. Proc Natl Acad Sci U S A. 2012;109(23):9149-9154.
145. Lim JH, Stafford BK, Nguyen PL, et al. Neural activity promotes long-distance, target-specific regeneration of adult retinal axons. Nat Neurosci. 2016;19(8):1073-1084.
146. Sanes JR, Masland RH. The types of retinal ganglion cells: current status and implications for neuronal classification. Annu Rev Neurosci. 2015;38:221-246.
147. Duan X, Qiao M, Bei F, Kim IJ, He Z, Sanes JR. Subtype-specific regeneration of retinal ganglion cells following axotomy: effects of osteopontin and mTOR signaling. Neuron. 2015;85(6):1244-1256.
148. Chang EE, Goldberg JL. Glaucoma 2.0: neuroprotection, neuroregeneration, neuroenhancement. Ophthalmology. 2012;119(5): 979-986.
149. Levin LA, Crowe ME, Quigley HA, Lasker/IRRF Initiative on Astrocytes and Glaucomatous Neurodegeneration Participants. Neuroprotection for glaucoma: requirements for clinical translation. Exp Eye Res. 2017;157:34-37.
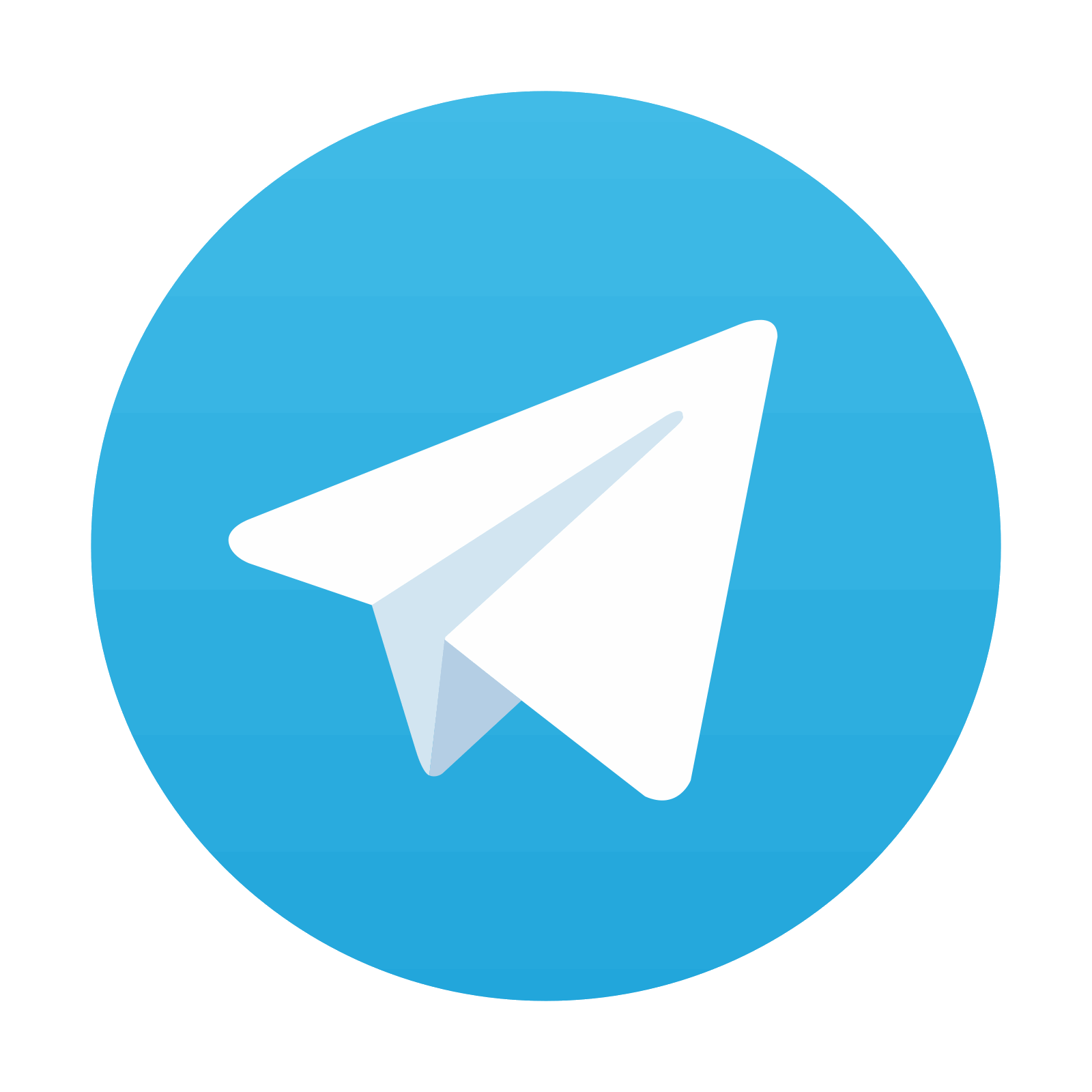
Stay updated, free articles. Join our Telegram channel
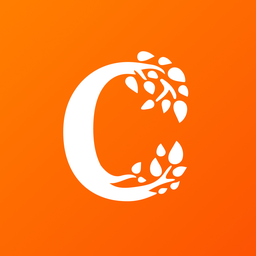
Full access? Get Clinical Tree
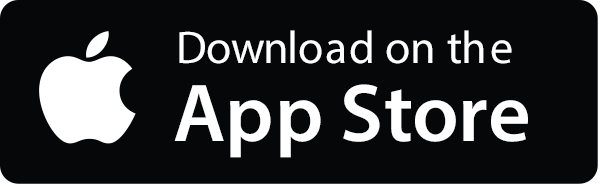
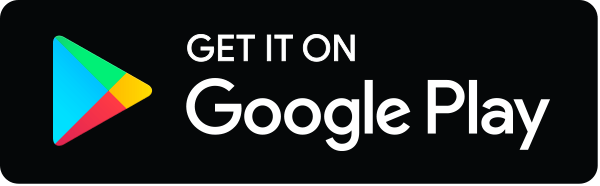