Nasal and Paranasal Sinus Physiology
Summary
The nose is the entry point to the respiratory tract, where over 10,000 to 20,000 L of air are exchanged daily. Among the most important physiologic functions of the nose are the conditioning (warming and humidification) and filtration of the inspired air and its role as the end organ for the sense of smell. It also fulfills an important defense function, as the nasal mucosa is the first site of interaction between the host tissue and foreign invaders (i.e., bacteria, allergens, and chemical and other stimuli). The pattern and dynamics of inspiratory and expiratory nasal airflow and how they interact with the nasal epithelium are essential to these important physiologic functions. They are highly influenced by many factors, such as the anatomy and microstructure of the nasal cavity, the functions of nervous system, and the vasculature, secretory tissue, and mucociliary of the nasal mucosa.
Physiologic Function of the Nasal Airflow
The special anatomical structure of the nasal cavity, with turbinates protruding from each lateral wall, is critical to the pattern and physiologic function of the nasal airflow. Individual aspects of the nasal cavity, such as the geometry and flow rate, collectively affect nasal function, for example, the filtration of foreign particles, by bringing inspired air into contact with mucus-coated walls, humidifying and warming the air before it enters the lungs, and acting as the end organ for the sense of smell.
The inspiratory nasal airflow for an adult can range from 5 to 12 L/min for calm breathing and increases to 40 L/min for physical exercise, with extreme airflow rates as high as 150 L/min.1 With such a range of flow rates, the Reynolds number (Re) can range from 4 × 102 to 1.2 × 104. Turbulent flow typically happens when Re is larger than 2 × 103, or 30 L/min (flow rate), inside a straight pipe. However, the onset of turbulence may occur at a lower Re in the complex geometries of the respiratory tract.2 For example, with nasal obstruction, the cross section of the nasal cavity is decreased, which increases local flow rates and induces turbulence.
Note
Individual aspects of the nasal cavity, such as the geometry and flow rate, collectively affect nasal function, for example, the filtration of foreign particles, by bringing inspired air into contact with mucus-coated walls, humidifying and warming the air before it enters the lungs, and acting as the end organ for the sense of smell.
In the normal nose, the bulk of airflow streamlines traverse the common meatus between the inferior and middle turbinates in a relatively vortex-free flow. The highest air speed is found in the anterior and middle airway, between the inferior and middle turbinates and the septum, and especially between the internal nasal valve and the anterior ends of the turbinates.1 The dynamics of airflow and changes in airflow velocity are related to the physiology of the nose. The greatest airflow occurs through those areas of the nose involved in air conditioning, and the slow velocity of airflow over the olfactory area protects this sensitive area of the nose from the damaging effects of inspired air, yet still allows sampling of the air.3
Assessment of Nasal Airflow
Impairment of the physiologic nasal airflow pattern or dynamics leads to the common clinical problem of subjective nasal obstruction, which is difficult to quantify by clinical examination; therefore, objective assessment of the nasal airway is critical to rhinologic research. A significant goal of applied clinical physiology is to provide objective correlates of subjective symptoms or sensations. It is important to characterize pathologic nasal obstruction and to distinguish it from the periodic nasal cycle, which is a normal variation in nasal patency.
Objective Measurements
Common methods used to objectively measure nasal patency and resistance are rhinomanometry and acoustic rhinometry. Rhinomanometry is a well-established technique that directly determines nasal airflow and airflow resistance. Acoustic rhinometry is a newer technique that acoustically measures the nasal cross-sectional area and the internal nasal cavity volume, thereby assessing structural pathologies of the nasal passage. Both techniques are complementary, and both provide accurate outcome assessments of the nasal airway. Details of these measurements are presented in Chapter 6.
Evaluation of both subjective complaints and objective measurements of nasal obstruction are essential for research. Subjective assessment of nasal obstruction is commonly performed using a symptom severity score rating or a visual analogue scale (VAS). However, subjective complaints and objective measurements of nasal obstruction are not always concordant. For example, increased subjective nasal obstruction is not always accompanied by objective increased nasal airway resistance, decreased nasal peak flow, or reduced acoustic rhinometry values. Suzina et al4 showed this dissociation when they demonstrated that active anterior rhinomanometry is a sensitive test of obstruction, but is not specific for the detection of abnormalities in nasal airway resistance that create symptomatic nasal obstruction. Similarly, in another study,5 the effect of 4 weeks of treatment with fluticasone propionate on symptoms of nasal obstruction in patients with perennial allergic rhinitis (PAR) was evaluated by using both a VAS and acoustic rhinometry measurements before and after the treatment. There was a significant improvement in the posttreatment VAS, as well as a significant increase in nasal cavity volume and minimum cross-sectional area (MCA). However, there was a poor overall correlation between the degree of subjective symptom improvement by VAS and that measured by acoustic rhinometry. This poor correlation between subjective and objective measurements could be due to the fact that the VAS records continuous ordinal data, whereas acoustic rhinometry measurements produce numerical data. Human sensing is complex and partially nonlinear; therefore, it is possible that small changes in nasal shape and in acoustic rhinometry measurements may result in large shifts in the VAS.
In some studies, better correlation between subjective and objective obstruction measurements can be demonstrated. In a nasal allergen challenge study in patients with PAR with house dust mite (HDM) allergy,6 a four-point subjective symptom score was compared with acoustic rhinometry results and demonstrated a significant inverse relationship between symptom score severity and MCA (r = − 0.568; P < .001). With subjective symptom scores of 0 (MCA 0.73 ± 0.22) and 1 (MCA 0.63 ± 0.29), there was a wider range of MCA values, corresponding to the normal variation of nasal cavity dimensions. The MCA range was less with more severe nasal obstruction (score 2, MCA 0.33 ± 0.17; score 3, MCA 0.21 ± 0.14).
In Vitro Models Used in the Study of Nasal Airflow
Anatomical Models
Human nasal airflow can be studied using water and dye flowing through anatomically accurate acrylic models of human nasal passages. The models are cast from the nasal cavities of human cadavers. The relationship between intranasal geometry and turbulent airflow could be evaluated by examining the flow regimes (laminar, semiturbulent, or turbulent) at different flow rates. A study was able to show that flow regimes and principal pathways were highly variable, and the relative projection of the inferior turbinate was the only variable that significantly affected the flow rate at which the flow became turbulent.7 However, more prominent turbinates appear to laminate flow rather than to induce turbulence. Nostril orientation was moderately correlated with flow dynamics; more inferiorly directed nares produced turbulence at slower flow rates. Relative nasal valve area and nasal sill height were unrelated to turbulence in these models.7
Particle Image Velocimetry
Particle image velocimetry (PIV) is an optical method of flow visualization, in which fluid is seeded with tracer particles, which are assumed to faithfully follow the flow dynamics and whose speed and direction can then be measured. In a study, PIV was used to determine two-dimensional (2D) instantaneous velocity vector fields in parallel planes throughout a model of the nasal cavity that was subjected to a nonoscillatory flow rate of 125 mL/s.8 The model, which was fabricated from 26 computed tomography (CT) scans using rapid prototyping techniques, was a scaled replica of a human right nasal cavity. The resulting vector plots show that the flow is laminar and that regions of highest velocity are in the nasal valve and the inferior airway. The relatively low flow in the olfactory region appears to protect the olfactory bulb from particulate pollutants. Low flows were also observed in the nasal meatus, whose primary function has been the subject of debate.
Computational Fluid Dynamics Models
In an attempt to provide more objective and quantitative measures of nasal airflow patterns, computational fluid dynamics (CFD) has been adapted for studying nasal aerodynamics. CFD is a branch of fluid mechanics that uses numerical methods and algorithms (usually performed by high-power supercomputers) to analyze problems that involve fluid flows.
During the past 10 years, CFD studies in three-dimensional (3D) human nose models constructed from CT scans or magnetic resonance imaging (MRI) have simulated human nasal models9,10 ( Figs. 2.1, 2.2, and 2.3 ). As compared with rhinomanometry and acoustic rhinometry, CFD enables additional measurements of airflow passing through the nasal cavity that help visualize the physiologic impact of alterations in intranasal structures. Therefore, it becomes possible to quantitatively measure and visually appreciate the airflow pattern (laminar or turbulent), velocity, pressure, wall shear stress, particle deposition, and temperature changes at different flow rates, in different parts of the nasal cavity, and in the healthy and structurally abnormal nose11,12,13 ( Figs. 2.4 and 2.5 ).




The effects of both existing anatomical factors and postoperative changes can be assessed.14 It has been applied to evaluate its existence and physiologic functions in critical nasal areas, such as the olfactory slit and functional nasal valve region, with the presence of septal perforation and septal deviation ( Figs. 2.6, 2.7, 2.8, and 2.9 ) (see Nasal Valve section).13,15
From the CFD model of inferior turbinate hypertrophy, the total nasal cavity pressure during inspiration is found to be − 10 Pa in a healthy nose, but − 19 and − 33 Pa in moderate and severe obstructions, respectively. The negative nasopharyngeal pressure induced by this nasal obstruction could affect soft palate function and contribute to snoring and obstructive sleep apnea.9




Note
As compared with rhinomanometry and acoustic rhinometry, CFD enables additional measurements of airflow passing through the nasal cavity that help visualize the physiologic impact of alterations in intranasal structures. Therefore, it becomes possible to quantitatively measure and visually appreciate the airflow pattern (laminar or turbulent), velocity, pressure, wall shear stress, particle deposition, and temperature changes at different flow rates, in different parts of the nasal cavity, and in the healthy and structurally abnormal nose.11,12,13
Note
The negative nasopharyngeal pressure induced by this nasal obstruction could affect soft palate function and contribute to snoring and obstructive sleep apnea.9
In addition, eustachian tube function, important in the pathogenesis of middle ear disease, is affected by nasal obstruction. In this study, the authors observed a fourfold higher air velocity and negative pressure (− 35%) at the eustachian tube orifice.9
Olfaction also depends on appropriate airflow past the olfactory epithelium at the apex of the nasal cavity. In the normal nose,9 the inspiratory airstream in the upper nose is low volume with low velocity and wall shear stress and measureable durations for particle deposition, which could be the optimal condition for achieving good olfactory function.3
Note
In the normal nose,9 the inspiratory airstream in the upper nose is low volume with low velocity and wall shear stress and measureable durations for particle deposition, which could be the optimal condition for achieving good olfactory function.3
However, in cases of moderate and severe nasal blockage, higher velocities and shear stresses, with negligible particle deposition times, are observed high in the nasal cavity, including the olfactory groove. These changes in airflow pattern could explain the impairment of olfaction in patients with persistent nasal obstruction due to inferior or middle turbinate hypertrophy ( Fig. 2.10 ).
Nasal airflow simulations predict high wall shear stress along the anterior aspect of the inferior turbinate, the anteroinferior aspect of the middle turbinate, and within Little′s area. The airflow simulations indicate that the inferior and middle turbinates and Little area on the anterior nasal septum contribute significantly to nasal air conditioning. The concentration of wall shear stress within Little area indicates a desiccating and potentially traumatic effect of inhaled air that may explain the predilection for spontaneous epistaxis at this site.16

It can be argued that CFD studies are only simulations, and the predicted results are derived from complex calculations of the Navier-Stokes equation,17,18 which may not represent real-life conditions. In the CFD model simulations, air is routinely regarded as being incompressible, in order for fluid dynamic theories to be easily applicable. This assumption is acceptable, given that the pressure changes within the nasal cavity are small. Additionally, the aerodynamics of nasal airflow is complex due to the geometry of the nasal cavity. Unlike a smooth, incompressible tube, the nature of nasal airflow (laminar, turbulent, or semiturbulent) in different parts of the nasal cavity varies due to the changes in surface structures, which requires some mathematical smoothing to enable simulation. As computer processing improves, closer approximations to the precise nasal contours will be able to be even more accurately reflected in better 3D models. Already, however, the CFD models produce realistic models that agree with other methods of observing nasal airflow and produce consistent, understandable results.
In the future, CFD studies may be useful in clinical situations, such as predicting the degree of functional impairment due to injury or anatomical variations and could help in surgical planning of corrections for inferior turbinate hypertrophy and other nasal anatomical malformations. For this work, specialized CFD simulation software needs to be developed ( Figs. 2.11 and 2.12 ).
The Nasal Valve
The nasal valve (the primary airway resistor) is considered to be a site of major pathophysiologic concern. It occurs at the entrance of the piriform aperture, the region just anterior to the tip of the inferior turbinate.19 It is the narrowest region of the nasal passage, which determines the nasal resistance to airflow; therefore, the nasal valve is an important functional region of the nasal airflow.20



Previous studies have attempted to visualize the nasal valve region using endoscopy, acoustic rhinometry, and CT,21 but none have been able to illustrate the relationship between form (anatomy) and function (physiology) of the nasal valve. Using a CFD model, it can be shown that in the healthy nose, the inspiratory airflow with the highest air speed is found in the anterior and middle airway, between the inferior and middle turbinates and the septum, and especially between the internal nasal valve and the anterior ends of the inferior turbinate.9 However, it disappears in severe nasal obstruction due to the changes of the airflow pattern, such as a lower velocity (50%) of inspiratory airflow, decreased wall shear stress (70%), and reduced negative pressure (80%) at the original nasal valve location9 (see Figs. 2.6 and 2.10 ).
In a healthy nose, the nasal valve creates a zone of high turbulent kinetic energy, high velocity, high negative pressure, and high wall shear stress. The nasal valve also changes the direction of the inspiratory airstream from the vestibule and directs the bulk of airflow around the inferior turbinate. The aerodynamics of the airflow changes significantly from a relatively laminar profile at the vestibule to having high turbulent kinetic energy anterior to the head of the inferior turbinate.
This is critical to facilitating mucosal contact for heating/cooling, humidification, and filtration of inspired air. This simple structure, in conjunction with turbinate blood flow, gland function, and mucus transport, may explain directly the major physiologic function of the nose in conditioning inspired air.
Note
The nasal valve (the primary airway resistor) is considered to be a site of major pathophysiologic concern. It occurs at the entrance of the piriform aperture, the region just anterior to the tip of the inferior turbinate. It is the narrowest region of the nasal passage, which determines the nasal resistance to airflow; therefore, the nasal valve is an important functional region of the nasal airflow.
Note
In a healthy nose, the nasal valve creates a zone of high turbulent kinetic energy, high velocity, high negative pressure, and high wall shear stress. The nasal valve also changes the direction of the inspiratory airstream from the vestibule and directs the bulk of airflow around the inferior turbinate. The aerodynamics of the airflow change significantly from a relatively laminar profile at the vestibule to having high turbulent kinetic energy anterior to the head of the inferior turbinate.
The Nasal Cycle
Airflow through both nasal passages is usually asymmetrical because of spontaneous congestion and decongestion of the nasal venous sinuses at the anterior end of the inferior turbinate and nasal septum in the nasal valve region.20 This nasal airflow asymmetry alternates over a period of 2 to 4 hours and is usually referred to as the “nasal cycle.”22 It was reported that the spontaneous nasal cycle of airflow resistances, which alternates between nasal cavities of seated subjects, persists in healthy young adults standing, supine, and laterally recumbent.23 In addition, the persistence of the cycle was recorded throughout 24-hour periods of unrestricted light activity, rest, and recumbency. Recumbency was found to augment amplitude of the cycle, yet resistance of the combined nasal cavities showed little change to accompany daytime activity or nocturnal sleeping postures.
Most subjects are completely unaware of any cyclic changes in nasal airflow because the total resistance to airflow remains relatively constant due to a reciprocal relationship between the nasal passages. The nasal cycle may not always be detectable due to minor reciprocal variation unrelated to time, or it may not be reciprocal at all between the two sides of the nose.24 The amplitudes of nasal patency fluctuation increase in subjects with allergic rhinitis,24 but they can totally disappear after application of a nasal decongestant (e.g., 1% oxymetazoline).
The physiologic role of the nasal cycle is not completely understood. Studies have shown that a fenestrated endothelium is present in nasal venous sinusoids and that the nasal cycle is increased during periods of nasal infection. Additionally, clinical observations have linked nasal fluid production to the decongestion of nasal venous sinusoids. As a result, the hypothesis that the nasal venous sinusoids may participate in respiratory defense mechanisms by production of plasma exudate has been put forward. Within the framework of this hypothesis, it has been proposed that the periodic congestion and decongestion of nasal venous sinusoids may provide a pump mechanism for the generation of plasma exudate and that this mechanism is an important component of respiratory defense.24
Conditioning of Inspired Air
The nasal cavity is able to warm and humidify inspired air optimally, which is essential for ideal pulmonary gas exchange, to avoid desiccation and adhesion of the alveolar capillary bed.25 A CFD study showed that anterior regions of the nasal cavity, especially around the inferior turbinate, are important for this function during inspiration.14 In a healthy nose, the mucosa is able to heat inspired air from 5 to 34°C (41–93.2°F). However, this warming function is impaired in a nasal model with total inferior turbinectomy or a large reduction in the turbinate head.
Various methods have been developed for measuring the temperature and relative humidity of inspired air before and after nasal conditioning in humans. However, the complex 3D anatomical structure of the nose makes it impossible to perform detailed in vivo studies on intranasal heating and humidification within the entire nasal airway applying various technical setups. A systematic review by Keck and Lindemann found that the main problem of in vivo temperature and humidity measurements is a poor spatial and time resolution.26 Therefore, in vivo measurements are feasible only to provide single temperature values, as the nose is not entirely accessible. Data on the overall performance of the nose are based only on a single measurement within each nasal segment.
Note
In a healthy nose, the mucosa is able to heat inspired air from 5 to 34°C (41–93.2°F). However, this warming function is impaired in a nasal model with total inferior turbinectomy or a large reduction in the turbinate head.
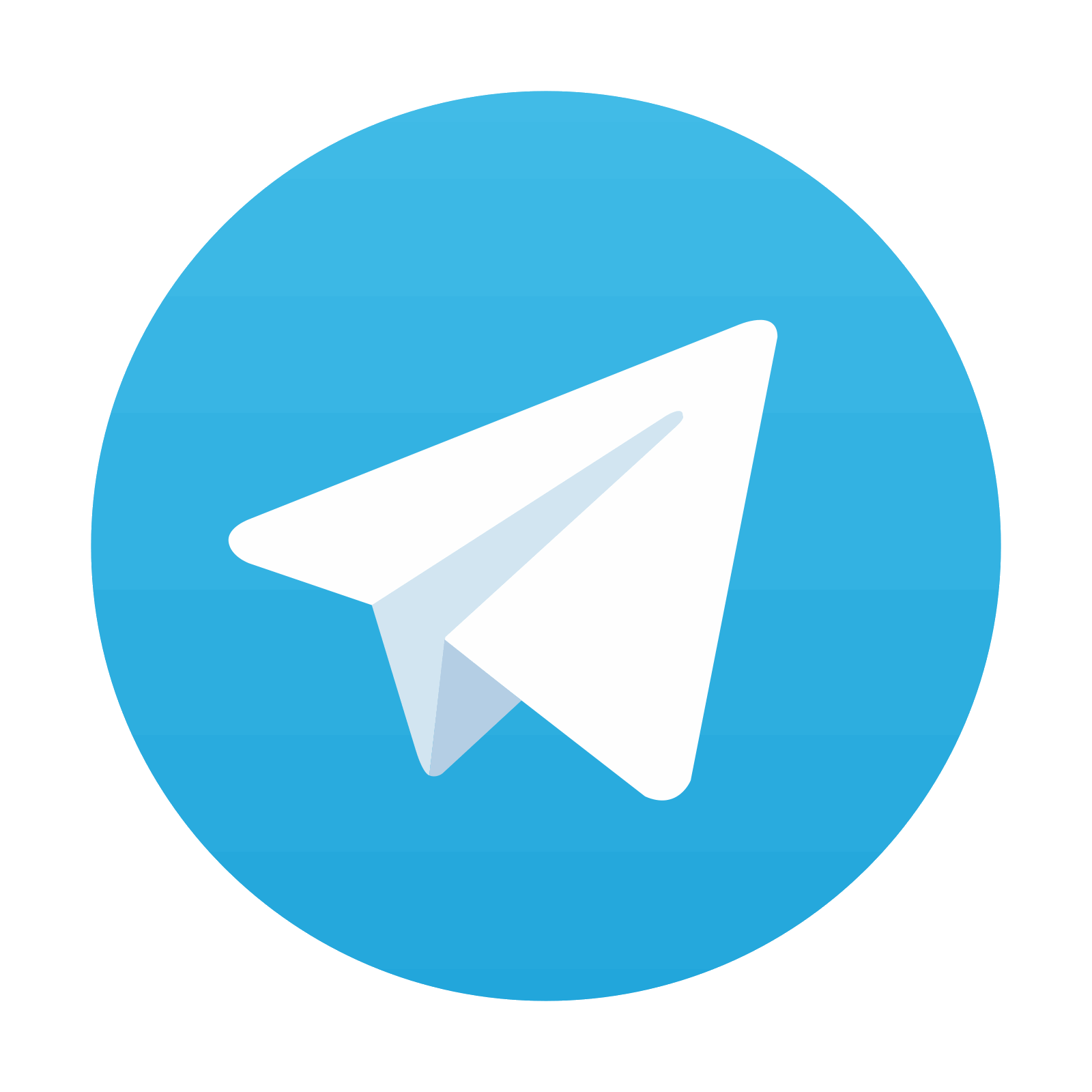
Stay updated, free articles. Join our Telegram channel
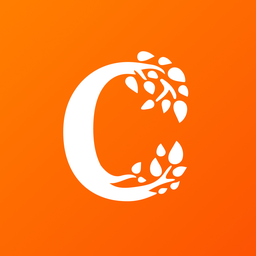
Full access? Get Clinical Tree
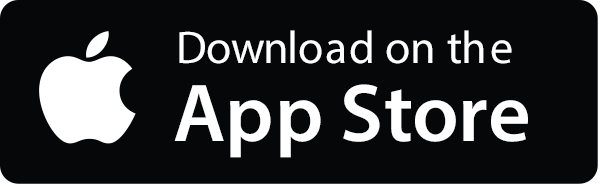
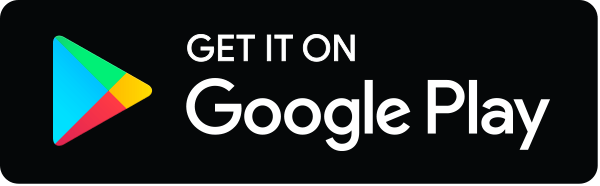
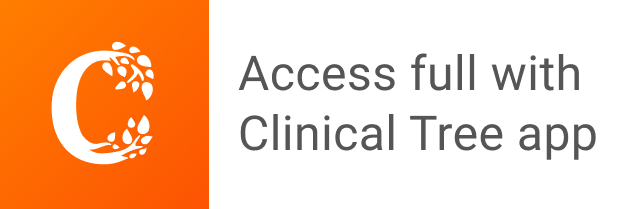