Purpose
To review the fields of nanotechnology and nanomedicine as they relate to the development of treatments for vision-threatening disorders.
Design
Perspective following literature review.
Methods
Analysis of relevant publications in the peer-reviewed scientific literature.
Results
Nanotechnology involves the creation and use of materials and devices at the size scale of intracellular structures and molecules and involves systems and constructs on the order of <100 nm. The aim of nanomedicine is the comprehensive monitoring, control, construction, repair, defense, and improvement of human biological systems at the molecular level, using engineered nanodevices and nanostructures, operating massively in parallel at the single cell level, ultimately to achieve medical benefit. The earliest impact of nanomedicine is likely to involve the areas of biopharmaceuticals (eg, drug delivery, drug discovery), implantable materials (eg, tissue regeneration scaffolds, bioresorbable materials), implantable devices (eg, intraocular pressure monitors, glaucoma drainage valves), and diagnostic tools (eg, genetic testing, imaging, intraocular pressure monitoring). Nanotechnology will bring about the development of regenerative medicine (ie, replacement and improvement of cells, tissues, and organs), ultrahigh-resolution in vivo imaging, microsensors and feedback devices, and artificial vision. “Regenerative nanomedicine,” a new subfield of nanomedicine, uses nanoparticles containing gene transcription factors and other modulating molecules that allow for the reprogramming of cells in vivo.
Conclusions
Nanotechnology already has been applied to the measurement and treatment of different disease states in ophthalmology (including early- and late-stage disease), and many additional innovations will occur during the next century.
Nanotechnology involves the creation and use of materials and devices at the size scale of intracellular structures and molecules and involves systems and constructs on the order of <100 nm. (For comparison, a typical man is 1.6 meters [1.6 billion nanometers (nm)] tall; an erythrocyte is 7 micrometers [7 × 10 3 nm] wide; a strand of deoxyribonucleic acid [DNA] is 2 nm wide.) Nanotechnology provides an important new set of tools for the diagnosis and treatment of ocular diseases. Miniaturization of devices, chip-based technologies, and increasingly sophisticated novel nano-sized materials and chemical assemblies already provide novel tools that are contributing to improved healthcare in the 21st century. Exciting new developments in the field of nanotechnology (cf. recent reviews ) are now impinging directly on ophthalmology.
Two examples illustrate the power of nanotechnology: information storage and computation. Regarding information storage, Richard Feynman, who conceived of the field of nanotechnology, calculated that it was possible to write the entire 24 volumes of the Encyclopedia Britannica on the head of a pin. Furthermore, he deduced that if one did not simply etch the letters onto the surface of the pin but instead used the interior of the material also, one could fit all the information that man had accumulated up to December 1959 (estimated at 10 15 bits) in a cube of material 1/200 inch wide, approximately the size of a piece of dust. Today, through the science associated with the precision assembly of matter, nanotechnology, storage densities of 10 11 bits per cm 2 have been demonstrated, which is close to achieving Feynman’s vision. This achievement is not surprising since each cell in our body stores an enormous amount of information. A retinal pigment epithelial cell has a diameter of approximately 4 × 10 −4 inches, and each cell stores the entire blueprint to create a human in DNA molecules (3 billion chemical base pairs, ∼25 000 genes) in which approximately 50 atoms are used for 1 bit of information about the cell.
Regarding computation, Feynman also noted that biological systems do not simply store information, they create measurable outputs. The human brain has the capacity to make judgments, eg, recognize a person’s face (even if shown at different distances, under different lighting conditions, at different angles), or play chess. According to Feynman, logic theory implied that if computers could have as many computational elements as our brains, they could make judgments as well. Because the size of our computational elements was small, we could fit more of them into our “bone box” than could be fit into a computer. Feynman’s prediction seems to have been correct at least in part. Today, sophisticated facial recognition can be accomplished well with a powerful laptop computer (vs poorly in 1959, with a much larger computer), because of the development of microprocessors (as well as the development of sophisticated software). To defeat a grand master at chess, however, a supercomputer is needed. A Cray XT5 supercomputer uses ∼40 kW power/cabinet, and each cabinet measures ∼81 × 23 × 57 inches (larger than a refrigerator) and weighs ∼1530 pounds ( http://www.cray.com/downloads/CrayXT5Blade.pdf ). It is remarkable that the “computer” in our cranium does not require the amount of rare elements, generate the heat, or have the energy requirements of a supercomputer. Thus, our cognitive capacities are one demonstration that it is possible to develop nano-scale mechanical systems that create complex, measurable outputs.
Nanotechnology and Nanomedicine
The aim of nanomedicine is the comprehensive monitoring, control, construction, repair, defense, and improvement of human biological systems at the molecular level, using engineered nanodevices and nanostructures, operating massively in parallel at the single cell level, ultimately to achieve medical benefit. Individual atoms and molecules can be manipulated to form microscopic tubes, spheres, wires, and films for specific tasks, such as generating electricity or transporting drugs in the body (see below for examples). The application of nano-scale technologies to the practice of medicine will alter profoundly our approach to diagnosis, treatment, and prevention of disease.
General principles of nanotechnology as applied to nanomedicine include:
- •
Biomimicry: using the approach that cells use to direct molecules within a cell and/or direct molecules/machines to the proper cells in the body
- •
Size and location drive biocompatibility and biological efficacy
- •
Engineer feedback control into therapeutic systems (eg, therapeutic gene synthesis)
- •
Molecules as machines: engineer molecules to perform specific physical tasks (eg, open ion channels) to alter cell and organism behavior
- •
“ Pseudointelligence ” resulting from intelligent design, eg, self-assembly of extracellular matrix molecules
- •
Highly interdisciplinary undertaking: biology, engineering, chemistry, physics
Some Properties of Nanomachines
Physical Properties
At the nanolevel (ie, the size scale of intracellular structures and molecules), materials acquire properties that seem surprising to us but that are predicted based on the principles of quantum physics. For instance, carbon becomes stronger than steel, gold melts at room temperature, and aluminum becomes highly explosive. Quantities such as weight and inertia are of relatively little importance. The strength of a given material is thus proportionately greater as the size diminishes.
Inhomogeneity of materials (eg, metals vs plastics) might limit their utility. Magnetic properties on a very small scale are not the same as on a large scale. Lubrication is not needed if the machine is small enough because heat loss is rapid (attributable to the large surface area–to-volume ratio). Some of these properties produce unexpected results. For example, rapid heat loss might prevent gasoline from exploding, which would make a nano-scale internal combustion engine impossible.
The influence of gravity on the function of true nanomachines probably is negligible because their mass is that of atoms (F g = Gm A m B /r ). (On the other hand, the distance between the elements is in nanometers.) Because of Van der Waals forces, parts of nanomachines might adhere to each other, which might not be desirable. Electrical resistance may be very large with nanocircuits (a feature that might be useful in biological systems). However, if one is working on the scale of atoms, then circuits might not be needed, and quantized energy levels might be manipulated for energy transfer according to the laws of quantum mechanics. (This property is exploited in some nanoparticle-based imaging technologies that we will consider.) Another problem with nanocircuits is the inverse relationship between the size of the device and the amount of noise generated (Hooge’s rule). (The development of stacked graphene sheets may provide a solution to this problem and facilitate the development of circuits much smaller than those in conventional silicone-based computer chips. )
Nanotubes and nanofibers are nanostructures with very large axial ratios. (Axial ratio is the ratio of the magnitude of the axes [eg, length and width in the case of a 2-dimensional object], the greater being divided by the lesser.) Synthetic methods exist to alter the length-to-volume ratio and thus the physical properties of the material. Conductivity, for example, is enhanced as the diameter of semiconducting nanotubes is reduced.
A key property of nanomaterials is that they are surface-rich objects in relation to volume. This notion is easy to visualize by a graphic analogy. A baseball filled with membrane surface–active enzymes would have only a minimal number of these extending to the surface of the ball, so the vast majority would be inactive. A pea filled with enzymes would have a greater proportion extending to the surface. A sphere 100 nm in diameter would have the majority of the enzymes it contains in an active state. Interestingly, this property does lead to a situation of extremely tight cell surface real estate in terms of attaching molecules to the nanoparticle surface. Vacancy-engineered mixed–valence state cerium oxide (CeO 2 ) nanoparticles (nanoceria particles) illustrate well the biologically useful properties that materials can develop at the nano scale. Alteration in the oxidation state of CeO 2 creates defects in its lattice structure via loss of oxygen or its electrons. As their size decreases, nanoceria particles demonstrate formation of more oxygen vacancies in the crystal structure. Chen and associates posited that engineered nanoceria particles can scavenge reactive oxygen intermediates because the large surface area–to-volume ratio at 5 nm diameter enables CeO 2 to regenerate its activity and thereby act catalytically. (Unlike nanoceria particles, most free-radical scavengers require repetitive dosing.) Chen and associates demonstrated that intravitreal injection of nanoceria particles prevents light-induced photoreceptor damage in rodents, even if injected after the initiation of light damage ( Figure 1 ). Vacancy-engineered nanoceria particles may function as a highly effective treatment for conditions associated with oxidative damage such as age-related macular degeneration (AMD), diabetic retinopathy, and retinitis pigmentosa.
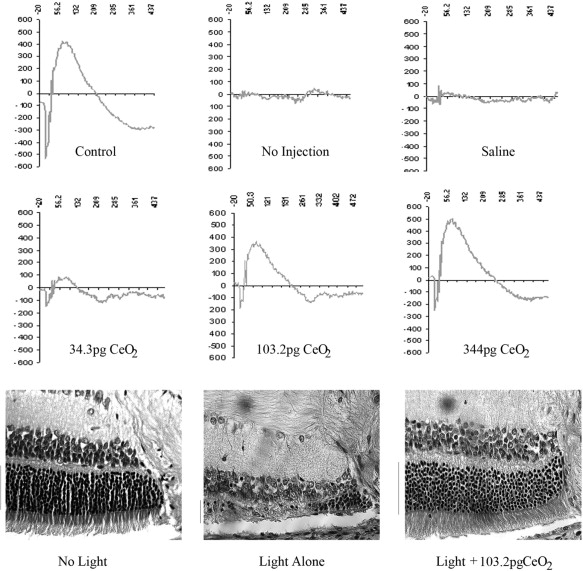
Manufacture
At each step in the process of manufacturing smaller and smaller machines, one must improve the accuracy of the equipment. If devices are built on the scale of 5 to 10 atoms, then it should be possible to mass-produce them such that they are perfect copies of each other. Perhaps one partial demonstration of the validity of this notion is the physical appearance of “identical” twins arising from the same zygote. (However, the process is not perfect! ) One useful outcome of a “nanomanufacturing” process is that the material costs of billions of these machines would be minimal, since each is so small that minimal material is used. (It may be of interest to note that the total monetary value of the elements in our body and our skin has been estimated at $4.50, which is consistent with the preceding conjecture.) The fact that we can heal from surgical and accidental trauma illustrates that nanomachines can have the capacity to repair and build themselves. Indeed, most nanoparticle structures are self-assembling under the proper thermodynamic conditions. This feature allows for production of a high number of virtually identical nanostructures.
Some properties of nanomachines are illustrated by the design and manufacture of an axon surgery platform. Using microtechnology, electrokinetic axon manipulation (ie, dielectrophoresis), and cell fusion (ie, electrofusion), Sretavan and associates have developed a paradigm of direct axon repair involving the substitution of damaged axon regions with healthy segments from donor axons. This multidisciplinary group developed a multifunctional axon surgery platform that is ∼1 mm 3 ( Figures 2, 3 ). The cutting device consists of a silicon nitride knife with an ultrasharp knife edge mounted onto a silicon-based compliant knife suspension ( Figure 2 ). The knife edge’s radius of curvature (∼20 nm) is similar to the diameter of a single microtubule. The knife is manufactured from a silicon nitride membrane and, effectively, is transparent, which permits visualization of axons during the cutting procedure. The mechanical compliance of the suspension can be varied within a range to deliver sufficient force for cutting various tissues (eg, single axons) or for harvesting specific cell populations from histologic tissue sections. The authors envision future improvements such as sensors as well as force-generating actuation mechanisms that automatically will deliver a controlled cutting stroke, and they indicate that both piezoelectric and thermal expansion actuation mechanisms can deliver forces in the range needed for axon cutting. (One might also consider using femtosecond laser surgery for axotomy. ) Thus, it may be possible to develop a microcutting device with on-board sensing and actuation that can function as a semiautonomous instrument, requiring only initiating commands from the surgeon. Important limitations to the practical application of this invention remain. The authors estimate, for example, that ∼20 seconds are required to repair a single axon using dielectrophoresis and electrofusion. Cutting and fusing multiple axons simultaneously might enable relatively rapid repair of several thousand axons.
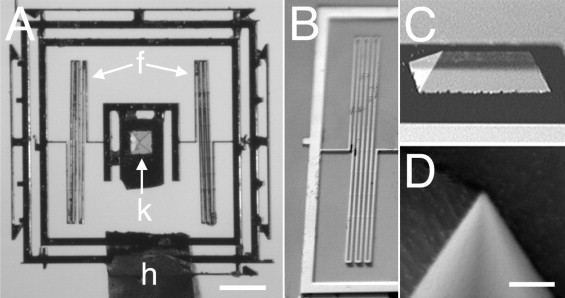
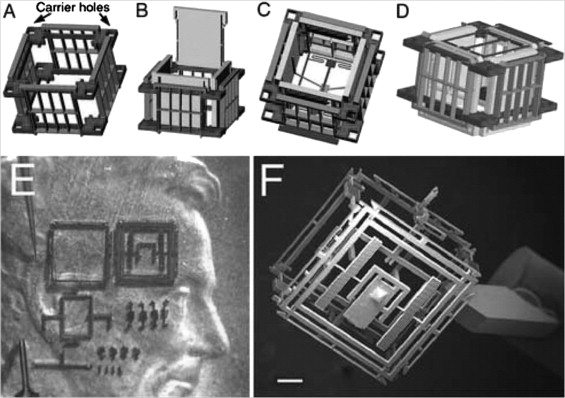
Some Properties of Nanomachines
Physical Properties
At the nanolevel (ie, the size scale of intracellular structures and molecules), materials acquire properties that seem surprising to us but that are predicted based on the principles of quantum physics. For instance, carbon becomes stronger than steel, gold melts at room temperature, and aluminum becomes highly explosive. Quantities such as weight and inertia are of relatively little importance. The strength of a given material is thus proportionately greater as the size diminishes.
Inhomogeneity of materials (eg, metals vs plastics) might limit their utility. Magnetic properties on a very small scale are not the same as on a large scale. Lubrication is not needed if the machine is small enough because heat loss is rapid (attributable to the large surface area–to-volume ratio). Some of these properties produce unexpected results. For example, rapid heat loss might prevent gasoline from exploding, which would make a nano-scale internal combustion engine impossible.
The influence of gravity on the function of true nanomachines probably is negligible because their mass is that of atoms (F g = Gm A m B /r ). (On the other hand, the distance between the elements is in nanometers.) Because of Van der Waals forces, parts of nanomachines might adhere to each other, which might not be desirable. Electrical resistance may be very large with nanocircuits (a feature that might be useful in biological systems). However, if one is working on the scale of atoms, then circuits might not be needed, and quantized energy levels might be manipulated for energy transfer according to the laws of quantum mechanics. (This property is exploited in some nanoparticle-based imaging technologies that we will consider.) Another problem with nanocircuits is the inverse relationship between the size of the device and the amount of noise generated (Hooge’s rule). (The development of stacked graphene sheets may provide a solution to this problem and facilitate the development of circuits much smaller than those in conventional silicone-based computer chips. )
Nanotubes and nanofibers are nanostructures with very large axial ratios. (Axial ratio is the ratio of the magnitude of the axes [eg, length and width in the case of a 2-dimensional object], the greater being divided by the lesser.) Synthetic methods exist to alter the length-to-volume ratio and thus the physical properties of the material. Conductivity, for example, is enhanced as the diameter of semiconducting nanotubes is reduced.
A key property of nanomaterials is that they are surface-rich objects in relation to volume. This notion is easy to visualize by a graphic analogy. A baseball filled with membrane surface–active enzymes would have only a minimal number of these extending to the surface of the ball, so the vast majority would be inactive. A pea filled with enzymes would have a greater proportion extending to the surface. A sphere 100 nm in diameter would have the majority of the enzymes it contains in an active state. Interestingly, this property does lead to a situation of extremely tight cell surface real estate in terms of attaching molecules to the nanoparticle surface. Vacancy-engineered mixed–valence state cerium oxide (CeO 2 ) nanoparticles (nanoceria particles) illustrate well the biologically useful properties that materials can develop at the nano scale. Alteration in the oxidation state of CeO 2 creates defects in its lattice structure via loss of oxygen or its electrons. As their size decreases, nanoceria particles demonstrate formation of more oxygen vacancies in the crystal structure. Chen and associates posited that engineered nanoceria particles can scavenge reactive oxygen intermediates because the large surface area–to-volume ratio at 5 nm diameter enables CeO 2 to regenerate its activity and thereby act catalytically. (Unlike nanoceria particles, most free-radical scavengers require repetitive dosing.) Chen and associates demonstrated that intravitreal injection of nanoceria particles prevents light-induced photoreceptor damage in rodents, even if injected after the initiation of light damage ( Figure 1 ). Vacancy-engineered nanoceria particles may function as a highly effective treatment for conditions associated with oxidative damage such as age-related macular degeneration (AMD), diabetic retinopathy, and retinitis pigmentosa.
Manufacture
At each step in the process of manufacturing smaller and smaller machines, one must improve the accuracy of the equipment. If devices are built on the scale of 5 to 10 atoms, then it should be possible to mass-produce them such that they are perfect copies of each other. Perhaps one partial demonstration of the validity of this notion is the physical appearance of “identical” twins arising from the same zygote. (However, the process is not perfect! ) One useful outcome of a “nanomanufacturing” process is that the material costs of billions of these machines would be minimal, since each is so small that minimal material is used. (It may be of interest to note that the total monetary value of the elements in our body and our skin has been estimated at $4.50, which is consistent with the preceding conjecture.) The fact that we can heal from surgical and accidental trauma illustrates that nanomachines can have the capacity to repair and build themselves. Indeed, most nanoparticle structures are self-assembling under the proper thermodynamic conditions. This feature allows for production of a high number of virtually identical nanostructures.
Some properties of nanomachines are illustrated by the design and manufacture of an axon surgery platform. Using microtechnology, electrokinetic axon manipulation (ie, dielectrophoresis), and cell fusion (ie, electrofusion), Sretavan and associates have developed a paradigm of direct axon repair involving the substitution of damaged axon regions with healthy segments from donor axons. This multidisciplinary group developed a multifunctional axon surgery platform that is ∼1 mm 3 ( Figures 2, 3 ). The cutting device consists of a silicon nitride knife with an ultrasharp knife edge mounted onto a silicon-based compliant knife suspension ( Figure 2 ). The knife edge’s radius of curvature (∼20 nm) is similar to the diameter of a single microtubule. The knife is manufactured from a silicon nitride membrane and, effectively, is transparent, which permits visualization of axons during the cutting procedure. The mechanical compliance of the suspension can be varied within a range to deliver sufficient force for cutting various tissues (eg, single axons) or for harvesting specific cell populations from histologic tissue sections. The authors envision future improvements such as sensors as well as force-generating actuation mechanisms that automatically will deliver a controlled cutting stroke, and they indicate that both piezoelectric and thermal expansion actuation mechanisms can deliver forces in the range needed for axon cutting. (One might also consider using femtosecond laser surgery for axotomy. ) Thus, it may be possible to develop a microcutting device with on-board sensing and actuation that can function as a semiautonomous instrument, requiring only initiating commands from the surgeon. Important limitations to the practical application of this invention remain. The authors estimate, for example, that ∼20 seconds are required to repair a single axon using dielectrophoresis and electrofusion. Cutting and fusing multiple axons simultaneously might enable relatively rapid repair of several thousand axons.
Applications to Ophthalmology
The rapidly expanding field of nanomedicine promises revolutionary advances in the diagnosis and treatment of disease. The earliest impact of nanomedicine is likely to involve the areas of biopharmaceuticals (eg, drug delivery, drug discovery), implantable materials (eg, tissue regeneration scaffolds, bioresorbable materials), implantable devices (eg, intraocular pressure [IOP] monitors, glaucoma drainage valves ), and diagnostic tools (eg, infectious disease diagnosis, genetic testing, imaging, IOP monitoring). Nanotechnology will bring about the development of regenerative medicine (ie, replacement and improvement of cells, tissues, and organs), ultrahigh-resolution in vivo imaging, microsensors and feedback devices, and artificial vision. “Regenerative nanomedicine,” a new subfield of nanomedicine, uses nanoparticles containing gene transcription factors and other modulating molecules that allow for the reprogramming of cells in vivo.
Delivery of Drugs, Peptides, and Genes
Nanoparticles are colloidal carrier systems that can improve the efficacy of drug delivery by overcoming diffusion barriers, permitting reduced dosing (through more efficient tissue targeting) as well as sustained delivery ( Figure 4 ). These features are attractive for drug treatment of chronic conditions such as glaucoma, uveitis, or retinal edema (attributable to venous occlusion or choroidal neovascularization), as well as for treatment of intraocular tumors and other conditions associated with cell proliferation, such as capsular fibrosis after cataract surgery, ocular neovascularization, and proliferative vitreoretinopathy. C-60 fullerene-based antioxidants might even be used to treat conditions characterized by oxidative damage, eg, AMD, retinitis pigmentosa, diabetic retinopathy, and retinopathy of prematurity (ROP). (C-60 fullerenes are cage-like structures of carbon atoms in the form of a truncated icosahedron. ) Liposomes may have been the first nanoparticles used for pharmaceutical therapy, since a triglyceride emulsion (∼200-nm-diameter micelles) are used for parenteral nutrition. Nanoparticles have been used for extraocular tumor therapy. Nanoparticles might be helpful in managing corneal allograft rejection. Yuan and associates manufactured 300-nm-diameter rapamycin-loaded chitosan/polylactic acid nanoparticles and found that these nanoparticles extended median allograft survival by 17% in rabbits compared with aqueous rapamycin eye drops. Although topical chitosan particles were well tolerated in this study, intraocular chitosan nanoparticles may not be well tolerated. Zhang and associates demonstrated that experimental autoimmune uveoretinitis responded very well to intravitreal liposomal tacrolimus (mean diameter = 200 nm) with no side effects on retinal function or systemic cellular immunity.
Nanoparticles also can be used to deliver growth factors to cells. Intravitreal nanoparticle-mediated basic fibroblast growth factor delivery provides sustained retinal rescue in Royal College of Surgeons rats. Intravitreal glial cell-derived neurotrophic factor-loaded biodegradable (poly)lactic-co-glycolic acid (PLGA) microspheres provide sustained ganglion cell protection in rodent models of glaucoma. PLGA has been approved for human use by the US Food and Drug Administration (FDA).
Dendrimers are synthesized, highly branched polymers that have precisely controllable nano-scale scaffolding and nanocontainer properties, which in some sense mimic the properties of macromolecules such as DNA and ribonucleic acid (RNA). The diameter of poly(amidoamine) dendrimer ranges from 1.5 to 14.5 nm. Dendrimers have been explored as vehicles for controlled drug delivery, including cancer therapy, pilocarpine, and vascular endothelial growth factor (VEGF) inhibition. Marano and asssociates, for example, used a lipophilic amino acid dendrimer to deliver an anti-VEGF oligonucleotide into rats’ eyes with laser-induced choroidal new vessels (CNVs). The dendrimer-oligonucleotide conjugate inhibited CNV development for 4 to 6 months by up to 95%, whereas eyes injected with oligonucleotide alone showed no treatment benefit compared to vehicle-injected controls at these times. The dendrimer-oligonucleotide conjugate was well tolerated in vivo, with excellent biodistribution and no observable increase in inflammation-associated antigens. Ideta and associates used dendrimer porphyrin encapsulated by a polymeric micelle to treat laser-induced CNVs in rodents and found significant enhancement of photodynamic therapy (PDT) efficacy with less light energy required for CNV occlusion.
Viral vectors (eg, lentivirus, retrovirus, adenovirus) deliver genes efficiently but are associated with risks, eg, immunogenicity and insertional mutagenesis. Nonviral vectors (eg, polymers, lipids) and other methods (eg, electroporation, nucleofection) have high gene-carrying capacity, have low risk of immunogenicity, and have relatively low cost and greater ease of production. Nanoparticles can deliver genes efficiently to stem cells. Many of these nanoparticle systems can be thought of as completely “synthetic viruses” whose composition is well defined and completely controlled (unlike use of actual viral vectors). These synthetic viruses can use transactivating sequences that allow them to deploy the host cell machinery to manufacture therapeutic molecules in situ. Since these sequences can contain an upstream biomolecular control sensor, therapeutic molecules can be manufactured in situ under tight feedback control of these biosensors.
Electrostatic interaction of cationic polymers with negatively charged DNA/RNA molecules results in condensation of the material into particles several hundred nanometers in diameter, protection of the genes from enzymes, and mediation of cellular entry. Polypexes (complexes of cationic polymers and plasmid DNA) can have transfection efficiency comparable to adenoviral vectors. Polypexes are small (diameter <8 nm), have large vector capacity, are stable in nuclease-rich environments, and have relatively high transfectivity for both dividing and nondividing cells. For example, nanoparticles compacted with a lysine 30-mer linked to 10-kDa polyethylene glycol containing CMV-CFTR cDNA were used successfully in a phase I/II clinical trial for treatment of cystic fibrosis. However, some particles have low transfection efficiency, and the duration of gene expression can be short. In general, the toxicity of polypexes and nanoparticles reflects their chemistry.
Nanoparticles have been explored as a means for gene delivery in the diagnosis and treatment of ocular disease. Compacted DNA nanoparticles can be targeted to different tissues in the eye by varying the injection site (eg, intravitreal injection can target the cornea, trabecular meshwork, lens, and inner retina; subretinal injection can target the outer retina and retinal pigment epithelium [RPE]). Preliminary results demonstrate photoreceptor rescue in an animal model ( rds +/− ) of retinitis pigmentosa using compacted DNA nanoparticles (polypexes) containing the wild-type retinal degeneration slow ( Rds ) gene. Albumin-derived nanoparticles that deliver plasmids containing genes for the Flt receptor, which binds free VEGF, penetrate keratocyte cytoplasm and provide sustained inhibition of injury-induced corneal neovascularization. (Albumin has a high charged amino acid content, which facilitates its action as a carrier for charged drugs and oligonucleotides.) PLGA nanoparticles can deliver genes to RPE cells in vitro and in vivo with reasonable efficiency, rapidity, and safety.
Nanoscale-engineered cell substrata (eg, nanowires) and carbon nanotubes also can be used for gene and drug delivery. Microfabrication technology has been adapted to produce arrays of microneedles that can penetrate the sclera (or cornea) to deliver drugs ( Figure 5 ). Biocompatible film that serves as a carrier for drug-loaded nanoparticles might be used for sustained submacular drug delivery ( Figure 5 ).
Imaging and Diagnostics
The use of nanomaterials for biomedical imaging has been reviewed thoroughly. Polyamidoamine dendrimer prototypes may be useful as targeted diagnostic magnetic resonance imaging (MRI) contrast agents as well as for controlled drug delivery. Gold nanoparticles have been used to enhance tumor identification with computed tomography (CT) and also for colorimetric biosensing. The latter assay is based on the fact that the plasmon resonance frequency is a function of the average distance between the gold particles as well as their size, their shape, and the dielectric properties of their environment. (A plasmon is a quantum of plasma oscillation and can be viewed as the oscillation of free electron density against the fixed positive charges in a metal.) As a result, 5-nm gold nanoparticles are orange-red but turn blue-purple when aggregated to larger nanoparticles. (Colloids of gold nanoparticles impart vibrant colors to the stained glass windows of some Gothic churches.) A gold nanoparticle–receptor complex can bind to a cross-linking molecule. Following such binding, nanoparticle clustering occurs with an associated colorimetric change. Superparamagnetic iron oxide (SPIO) nanoparticles have been approved for use by the FDA as MRI contrast agents. Typically, an SPIO nanoparticle is composed of an iron oxide core coated with dextran, which renders it water soluble. For in vivo long-term imaging, the label is internalized by endocytosis or phagocytosis with or without an excipient. These particles can have diameters of 60 to 150 nm and can be visualized using MRI. SPIO-labeled stem cells have been visualized in patients with brain trauma. Limitations of this approach include the dilution of signal associated with cell replication and/or migration. Also, no information is provided regarding the state of differentiation of the transplanted cells. Cytotoxicity (eg, via iron-catalyzed generation of reactive oxygen species) might be a limitation of this approach. Quantum dots (Qdots) are an alternative labeling material. Qdots are light-emitting nanocrystals (2-10 nm) composed of atoms from groups II to VI (eg, CdSe, ZnSe) or III to V (eg, InP) of the periodic table. An advantage of Qdots over SPIO nanoparticles is that Qdots can be visualized with optical imaging (vs more complex MRI), including optical coherence tomography (OCT). In contrast to most organic dyes and fluorescent proteins, Qdots have durable fluorescence intensity, which helps one to distinguish the signal from background autofluorescence, and a broad excitation/narrow emission spectrum, which permits analysis of multiple cell targets with a single excitation wavelength. Qdots can be used to monitor survival, distribution, and differentiation of stem cells in vivo. Limitations can include signal dilution (attributable to cell proliferation and/or migration) as well as cytotoxicity. In some cases, toxicity might arise from oxidative degradation of Qdots with subsequent Cd release and mitochondrial damage. Coupling SPIO nanoparticles or Qdots to antibodies that recognize molecules such as components of the complement system or molecular constituents associated with AMD-induced changes in Bruch membrane might provide a means to image the biochemical and/or structural abnormalities associated with AMD. This capacity may be crucial for development of AMD treatments that target early molecular changes and early stages of the disease.
Nanowires are structures with a diameter of less than 100 nm and indefinite length. Semiconductor nanowires have unique electronic properties and sizes comparable with biological structures involved in cellular communication, thus making them promising nanostructures for establishing active interfaces with biological systems. Cells can be grown on nanowire arrays, which can measure electrical functions in different parts of the same cell. These techniques should be applicable to measuring functions such as electrical activity and contractility in neurons, cardiac cells, and muscle cells, as well as the effects of drugs on their function. Ophthalmic applications might include monitoring ganglion cell survival/physiology in glaucoma patients or photoreceptor/RPE survival/physiology in transplant recipients.
Micro- and nano-electromechanical systems (M/NEMS)-based engineering permits the construction of small diagnostic devices. A microfabricated implantable wireless passive IOP sensor, for example, has been developed using this approach. However, this device, whose surface area measures 4 × 2 mm 2 , must be implanted into the anterior chamber to function. Dresher and Irazoqui have developed a compact, ultra-low-power operational amplifier that can be used to measure IOP. The device has a power consumption of 736 nW, a chip area of 0.023 mm 2 , and output impedance of 69 Ω. It is envisioned that this device could be placed in contact with the choroid via minimally invasive surgery.
Regenerative Medicine
Regenerative medicine through M/NEMS-based tissue engineering is a promising approach for treating retinal degenerations. However, reconstruction of functional retinal tissue requires effective application of developmental biology principles and biomaterials sciences to control the complex relationship between transplanted cells, scaffolding material, and host tissue. Micro- and nano-electromechanical systems permit fabrication of devices using computer-aided design and by the repeated application of a number of procedures including: oxidation, photolithography, etching, diffusion, sputtering, chemical vapor deposition, ion implantation, and epitaxy. Control of features down to the submicron level is achieved readily, which permits controlled production of mechanical structures at length scales ranging from 100 nm or less to greater than 1 cm. Microfabrication and micromachining techniques have been integrated into biological studies. The ability to create complex microfabricated biomaterial substrates using these techniques provides important advantages over traditional biomaterial surfaces because of the ability to control surface microarchitecture, topography, and feature size. In an engineered environment, individual cell responses can be controlled utilizing structures also at the micro and nano scale to alter cellular attachment and motility, attenuate the foreign body response, simulate tissue organization, and promote cell differentiation.
As implied above, nanotechnology can be used to create an extracellular matrix (ECM). The ECM can alter cell behavior. Stem cells, for example, are prevented from exiting the mitotic cycle by environments called niches, which comprise cellular and noncellular elements (ie, ECM components). The ECM contains sites for cell adhesion as well as growth factors. Integrins are transmembrane proteins that mediate contact between cells and ECM proteins such as laminin and fibronectin. Integrin binding to ECM ligands results in integrin clustering, formation of focal adhesion complexes, modification of cytoskeleton organization, and activation of intracellular signaling that affects processes such as cell adhesion, proliferation, and differentiation. Physical features of the ECM that influence cell behavior and phenotype include the size, lateral spacing, surface chemistry, and geometry of ECM ligands. For example, titanium oxide (TiO 2 ) nanotube nanoscale spacing of 15 nm, which corresponds approximately to the diameter of an integrin extracellular domain, supports optimal cell differentiation for bone remodeling–associated cells ( Figure 6 ). Substrate topography and electrical conductivity modulate the differentiation of neuronal stem cells into specific neuronal lineages. Keratocyte shape, alignment, and migration are guided by nanotopography. These features might be altered to improve biointegration of prosthetic devices such as artificial corneas.
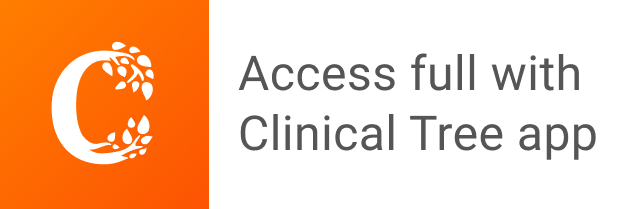