Definition and prevalence
Myopia is the most common human eye disorder in the world. For this refractive state, the retina is located behind the focal plane so that a concave (negative-power) lens is needed to move the focal plane back to the retinal plane, restoring a focused, clear image. Definitions of myopia vary, but generally an eye is considered myopic if a negative spherical equivalent correction of at least 0.50 diopters (D) is needed to restore emmetropia, the refractive state in which images are focused on the retina. Because of varying definitions, the reported prevalence of myopia varies. In the US adult population an estimated prevalence of 25–30% is supported by multiple studies. Females are reported to have an earlier onset and a slightly higher prevalence than males. US Asians and Hispanics have a higher prevalence than whites or African-Americans. Chinese and Japanese populations have very high myopia prevalence rates of > 50–70%. Ashkenazi Jews, especially orthodox Jewish males, have shown a higher prevalence than other white US and European populations. Comparative prevalence rates from different countries show considerable variability, but confirm that myopia affects a significant proportion of the population in many countries. Worldwide, there may be as many as one billion myopic individuals ( Box 55.1 ). Uncorrected myopia is an important cause of correctable low vision in developed and underdeveloped countries. A priority of refractive error correction is part of the World Health Organization’s global initiative, Vision 2020.
Why study myopia? Myopia has been and continues to be the most common human eye disorder worldwide, with increasing prevalence rates and health care costs. Severe degrees of myopia predispose those affected to a handful of ocular morbidities which can compromise sight and quality of life
Myopia is typically divided into two basic types. “Juvenile-onset” or moderate myopia (also called “simple” or “school” myopia) most often develops and progresses between the ages of 8 and 16 years, and generally does not require a correction stronger than −5 D. In contrast, “pathologic” or high-grade myopia usually begins to develop in the perinatal period, and is associated with rapid refractive error myopic shifts before 10–12 years of age due to axial elongation of the vitreous chamber.
Whether it occurs from continued progression of juvenile-onset myopia, or from early-onset high-grade myopia, a high level of axial myopia (spherical equivalent refractive correction of −5 D or greater) is a major cause of legal blindness in many developed countries. High myopia has a prevalence of 1.7–2% in the general population of the USA and is especially common in Asia. In Japan, high myopia reportedly affects 6–18% of the myopic population and 1–2% of the general population.
The public health and economic impact of myopia is considerable. Costs associated with optical corrections for adults were over US $26 billion in 2005 for glasses, contact lenses, and refractive surgery. Of this, at least 61% ($14.6 billion) was for myopic correction, and did not include costs for correcting myopia in children.
Ocular morbidities associated with myopia
Many investigators have reported on the association of high myopia with premature cataract development, glaucoma, severe retinal thinning with eventual retinal detachment (RD), and posterior staphyloma with retinal degenerative changes. High myopia is associated with progressive and excessive elongation of the globe, which may be accompanied by degenerative changes in the sclera, choroid, Bruch’s membrane, retinal pigment epithelium (RPE), and neural retina. Various fundoscopic changes within the posterior staphyloma develop in highly myopic eyes. These changes include geographic areas of atrophy of the RPE and choroid, lacquer cracks in Bruch’s membrane, subretinal hemorrhage, and choroidal neovascularization (CNV). Among these various fundus lesions, macular CNV is the most common vision-threatening complication of high myopia. Clinical and histopathologic studies have documented CNV in 4–11% of highly myopic eyes. Relative to emmetropic eyes, an approximately twofold increased risk of CNV was estimated for eyes with 1–2 D of myopia, a fourfold increase with 3–4 D, and a ninefold increase with 5–6 D. Poor visual outcome following CNV in myopic eyes is not uncommon, and often affects relatively young patients.
The risk of RD is estimated to be 3–7 times greater for persons with greater than 5 D of myopia, compared with those with a lower amount of myopia. Myopia of 5–10 D was associated with a 15–35-fold greater risk of RD relative to that associated with low levels of hyperopia. The lifetime risk for RD was estimated to be 1.6% for patients with myopia less than 3 D and 9.3% for those with more than 5 D. A subgroup with lattice degeneration and more than 5 D of myopia had an estimated lifetime risk of 35.9%. The prevalence of lattice degeneration increases with increasing amounts of myopia, as measured by axial length. Glaucoma was observed in 3% of individuals with myopia with axial lengths of less than 26.5 mm, in 11% with axial lengths between 26.5 and 33.5 mm, and in 28% of those with longer lengths.
Myopia is the most studied refractive error due to the high prevalence and the increased risk of associated blinding complications. Research pursuits include why and how myopia develops, and whether treatments can be developed to prevent this refractive error, or prevent progression to high amounts. A fundamental question is whether myopic development is a result of predetermined genetic or environmental factors such as excessive near work. Both appear to play a role in human myopia.
Human emmetropization process
Although myopia is highly prevalent, the majority of eyes are emmetropic in childhood. The normal postnatal development of emmetropia has been examined for clues about possible underlying mechanisms by which this is achieved. At birth ( Figure 55.1A ), the refractive distribution of human newborns is very broad. The mean is approximately 2 D of hyperopia, but the standard deviation is great and nearly 25% of newborns are myopic. The major determinants of the focal plane are the cornea and lens, while the axial length (vitreous chamber depth) determines whether the retina is located at the focal plane. At birth, the size, shape, and power of all are determined largely by inheritance, although conformational factors such as intrauterine environment and the bony orbits and eyelids can also influence eye shape and growth.

During the first postnatal weeks and months, the ocular components and refractive state undergo rapid changes. The corneal diameter of the infant is 9–10 mm compared to the adult size of 12 mm. Due to the steep curvature, corneal power averages 51 D at birth and flattens to approximately 44 D by 6 weeks of age.
Mutti et al found the average corneal power at 3 months of age to be 43.9 D. By 9 months it decreased to 42.8 D. Between 6 and 14 years of age, corneal power is stable. Lenticular power averages 34 D at birth and decreases to 28 D by 6 months of age, and to 21 D by adulthood. Mutti et al found the average lens power (Gullstrand–Emsley indices) showed a continual decline with age from 21.5 D at age 6 years to 19.8 D at age 14 years.
In addition to the changes in corneal and lens powers, the distribution of refractive errors narrows dramatically in the postnatal months ( Figure 55.1B ). In the infantile growth period the eyes grow from around 16 mm axial length at birth to an average of 19 mm at 3 months of age and over 20 mm by 9 months. During this time the axial length changes in a manner that moves the retina to the focal plane. As described by Mutti et al, “modulation in the amount of axial growth in relation to initial refractive error appeared to be the most influential factor in emmetropization of spherical equivalent refractive error.” Eyes that are initially hyperopic increase their axial length rapidly to move the retina to the focal plane. Eyes that are initially myopic have a slower axial elongation rate so that, as the cornea and lens powers decrease, the focal plane moves to the retina. The result of the controlled growth of the axial length is that nearly all eyes become emmetropic with the majority being slightly (0.5–1 D) hyperopic when measured with cycloplegia.
There is little change in refractive status in most eyes during the rest of childhood, even though there is continued change in anterior-chamber depth, lens power, and axial length. Because of the continued decreases in corneal and lens power during childhood, control of the axial elongation rate to maintain a match to the focal plane is needed until the eyes are fully mature. Refractive error distribution in the adult population has a narrow peak with most people between emmetropia and +1.0 D. This amount of hyperopia is readily compensated for by accommodation of the crystalline lens, so that most eyes are functionally emmetropic. The human eye normally maintains an axial length of within 2% of its optimal focal point. In an adult (~24 mm) eye, a deviation from optimal of 0.2 mm in axial length would produce a refractive error of more than 0.5 D.
Although the individual refractive components of the corneal and lenticular dioptric powers and anterior-chamber depth follow a bell-shaped (normal) distribution, several studies have shown that the refractive status of the eye is determined primarily by variation in axial length, which does not display a normal distribution. Spherical refractive error usually represents a mismatch between axial length and the combined dioptric powers of the cornea and lens. Moderate myopia results from a “failure of correlation” of these components where all components fall within normal limits, but are borderline high or low. For example, an eye with a relatively steep cornea and normal axial length could be myopic even though none of the ocular component dimensions is abnormal. Low myopia (smaller than 6 D) is usually the result of this lack of correlation. In children whose myopia is progressing the amount of progression is closely related to the increase in vitreous chamber length. Higher levels of myopia are due to “component ametropia,” in which the axial length exceeds normal values. “Correlational” and “component” myopia may have different genetic etiologies.
To some extent, narrowing of the distribution of refractive errors during the first postnatal months could be explained by the “passive proportional” growth of the eye. However, more eyes are emmetropic than would be expected from a random combination of the optical elements and the axial length. This has led to the suggestion that an active feedback mechanism coordinates the axial length with the optical elements to produce emmetropia. However, based on clinical observations, it was not possible to test this suggestion.
Animal models of emmetropization
In the 1970s, studies with animal models (primarily monkeys, chicks, and tree shrews ) demonstrated that an emmetropization mechanism exists. It has been shown in animals that this emmetropization mechanism normally controls the axial elongation rate of the eye to achieve and maintain a match of the axial length to optical power so that the photoreceptors are in focus for distant objects. Vision plays a critical role in this process ( Box 55.2 ).
Animal model studies of myopia provide the best source of biologically similar tissue types to test various eye growth paradigms. Their relationship to human myopia remains unclear however
Form deprivation myopia
Animal models of the emmetropization process began with “form deprivation myopia” (see recent reviews ). Form deprivation was initially produced by tarsorrhaphy or by the placement of a translucent diffuser over the eye, held in place by a goggle or mask. This eliminates higher spatial frequencies and decreases the contrast of the retinal image, while still allowing limited transmission of light to the retina. It is now recognized that form deprivation removes the visual feedback needed to guide the eye growth to an emmetropic state and to maintain emmetropia. Form deprivation during the juvenile postnatal period causes the vitreous chamber elongation of chicks, tree shrews, macaque monkeys, and other species. The axial growth continues from a standard, slightly hyperopic state extending past a set point that would produce emmetropia to become myopic. Degenerative retinal fundus changes typical of human myopia were noted in monkey and tree shrew. A decrease in choroidal thickness also occurs in the monkey and tree shrew, and is prominent in the chick. The “induced myopia” occurs only monocularly in form-deprived eyes, and not in the paired eye which serves as an untreated within-animal control. Thus, the myopia is clearly environmental in nature. The observation that eyelid closure myopia could not be induced in dark-reared animals further suggests that visual experience is required. Form deprivation myopia occurs consistently across species, including the grey squirrel, cat, and mouse.
Compensation for negative lenses
Form deprivation myopia demonstrates that the visual environment plays a role in establishing and maintaining emmetropia. Recognition that the emmetropization mechanism uses visual feedback to match the axial length to the focal plane emanates from studies that used negative-power (and positive-power) lenses to shift the focal plane of the eye. As shown in Figure 55.2B , a monocular negative lens shifts the focal plane posteriorly, away from the cornea. This consistently produces a compensatory increase in the axial elongation rate of the growing eye, such that the retinal location is shifted to match the shifted focal plane ( Figure 55.2C .) When measured with the lens in place, the refractive state matches the untreated fellow control eye. Thus, in compensating for the negative lens the eye is, in fact, restoring optical emmetropia.

With the lens removed, the eye is myopic ( Figure 55.2D ). Compensation can be quite accurate and negative lenses of different powers produce different axial elongation appropriate to move the retina to compensate for the lens power. Some strains of mice can develop negative lens-induced myopia, even though mice are not strongly dependent on vision. Fish ( Tilapia ), which are more vision-dependent, display this modulation characteristic.
Interestingly, for negative lens compensation to occur in animals, the lens must be worn almost constantly. Removing the negative lens and allowing normal unrestricted vision (or plano-lens wear) for as little as 2 hours per day is sufficient to block negative lens compensation in monkeys, tree shrews, and chicks.
Plus lenses
Plus lenses shift the focal plane anteriorly, converting an emmetropic eye into one that is myopic. In examining the effect of plus lenses in animal studies, it is important to note that they are applied to juvenile eyes that are still undergoing normal axial elongation. If the axial elongation rate is slowed below normal while the optical components continue to mature normally, the eye gradually becomes emmetropic while wearing the plus lens. With the lens removed it is hyperopic. Thus, to respond to a plus lens, the eye must not only be able to detect that it is myopic, but also be able to slow its elongation rate. In a young, growing chick eye, plus lens wear quickly causes a decrease of the elongation rate such that the eye becomes emmetropic while wearing the lens and hyperopic when the lens is removed. Even short exposures to myopic defocus have a potent slowing effect.
The response to plus lenses differs somewhat in monkeys and tree shrews. When plus lenses are applied to tree shrews that have achieved emmetropia, little effect is noted. However, if plus lenses are applied early in the emmetropization process in younger, hyperopic tree shrews and monkeys, the elongation rate of the eyes decreases, and emmetropia is maintained while wearing the lens. With lens removal, the eye remains hyperopic. The lack of a response to plus lenses in older tree shrews and monkeys may be due to an inability to slow axial elongation rate substantially below normal once the eyes have achieved emmetropia. The source of this difference in the chick is unknown, but may be related to different sclera composition in the avian eye relative to that of the mammalian. The chick eye sclera has an inner cartilaginous layer in addition to the fibrous sclera layer present in humans, monkeys, and tree shrews. Controlling the growth of the cartilage may be a more powerful way of controlling axial elongation than can be achieved with a fibrous sclera alone.
Recovery from induced myopia
Further evidence for active control of axial length growth comes from the effect of removing form deprivation or negative lens wear after an induced myopia has developed. In the several species that have been studied, “recovery” from induced myopia occurs. Restoration of unrestricted vision causes the axial elongation rate to decrease below normal. As the focal plane moves posteriorly due to continued corneal flattening and lens maturation the induced myopia dissipates. It has been suggested that recovery occurs for two reasons: first, the eye is myopic, and second, it is elongated relative to normal. These factors together produce a stronger response than is produced by plus lens wear alone. In animals with monocular induced myopia, the refractive state of the recovering eye eventually matches that of the untreated fellow control eye at emmetropia, suggesting that active guidance occurs moving towards a “target” of emmetropia. The emmetropization mechanism is thus capable of acting to control axial elongation to achieve emmetropia from the myopic, as well as the hyperopic, direction. The consistency of responses to form deprivation, negative lens wear, and recovery provides evidence that an active emmetropization mechanism exists and is conserved across phyla. Together with the rapid development in human infants of a very narrow refractive error distribution, these data argue strongly that an emmetropization mechanism exists in humans.
The emmetropization feedback loop
The signaling cascade
How does the retina control the axial elongation rate to match the retina to the focal plane and maintain that match throughout the juvenile developmental period? The broad outline of a feedback loop has emerged from studies of animal models. The starting place is the visual stimulus (1 in Figure 55.3 ). Although it is not precisely known what aspect of the images on the retina stimulates elongation, there is general agreement that hyperopic defocus (image plane behind the retina) serves as a stimulus for eyes to increase the axial elongation rate (“go” signal). Myopic defocus, or perhaps clear images projected on the retina, produces signals that slow axial elongation (“stop” signal). Defocus, or its absence, is detected by the retina (2 in Figure 55.4 ), presumably at the level of center-surround bipolar cells or later. As has been established by single-unit studies of retinal ganglion cells, defocused images produce weaker responses from cells with center-surround receptive fields than do sharply focused images. Thus, as the eyes move from target to target, with varying retinal image stimulation, focused images will produce large changes in the retinal cell activity level.


In chicks, a class of amacrine cells that contain glucagon are involved in sending a “stop” signal to slow the eye elongation rate of the eye. Without these cells, eyes elongate and become myopic. With them, and with additional glucagon administered intravitreally, eyes resist becoming myopic with form deprivation. It has also been reported that the protein apolipoprotein A1 provides a retinal “stop” signal in chicks.
Perhaps surprisingly, the emmetropization mechanism persists after sectioning of the optic nerve, or blocking the output from the retina to central visual structures with tetrodotoxin, leading to the conclusion that there is direct communication across the RPE and choroid (3 and 4 in Figure 55.3 ) to the sclera of the eye. The communication to the sclera must also be spatially local. When form deprivation or negative lens treatment is applied to half of the visual field (either nasal or temporal) using specially designed goggles, only the treated half of the eye elongates and becomes myopic. More surprisingly, the fovea is neither necessary nor sufficient to establish and maintain emmetropia. Macaque monkeys that underwent laser destruction of the entire macular region maintain emmetropia. Monkeys that wear form-depriving diffuser goggles with a clear central 30° to allow foveal vision develop form deprivation myopia.
How the visually derived signals pass through the RPE is unknown. It has been suggested that changes in retinal activity produce changes in ionic concentrations across the RPE that in turn affect the tissues of the choroid. This part of the emmetropization signaling cascade is under active investigation. Mertz and Wallman suggested that all-trans – retinoic acid in the choroid may serve as a signaling molecule to the sclera. Rada et al have suggested that ovotransferrin might also serve a similar role.
Visual response remodeling of the sclera
As the structural outer coat of the eye, the sclera controls the location of the retina. The sclera in eutherian mammals is a soft, but tough, fibrous viscoelastic connective tissue comprised of an extracellular matrix (ECM) produced by fibroblasts that are of neural crest origin. It consists of layers, or lamellae, composed of collagen (85–90% of the scleral total protein), associated proteoglycans, hyaluronan, and other extracellular proteins produced by the fibroblasts. Type I collagen is the primary subtype (>99%) but types III, V, and VI are present in tree shrew sclera. Unlike the corneal stroma, the diameter and spacing of the collagen fibrils vary widely, so that the sclera is not transparent. In addition to collagen, elastic fibrils comprised of elastin and laminin have been reported in the human sclera. The proteoglycans aggrecan, biglycan, and decorin are also present in the adult human sclera and in tree shrew sclera. Austin et al suggested that another proteoglycan, lumican, is important in the formation of scleral collagen fibrils.
Glycosaminoglycans (GAGs) constitute a small (~0.2%), but potentially important, fraction of the scleral dry weight. Functionally, GAGs have been suggested to stabilize the spacing of collagen fibrils and to affect the hydration levels of ECM.
In addition to these structural components, the sclera contains several growth factors (e.g., transforming growth factor-ß, fibroblast growth factor-2) as well as degradative enzymes (matrix metalloproteinases and tissue inhibitors of metalloproteinases). A simplified scleral structure is provided in Figure 55.4 .
After completion of the infantile growth period, there is little evidence in mammals for continued scleral remodeling. Rather than modulating cartilage growth (as occurs in chicks ), the visual environment controls remodeling of the scleral ECM. This discussion will focus on mammalian sclera, with particular emphasis on tree shrew because it has been the subject of considerable research.
When “go” signals reach the sclera, changes in expression for some genes occur (5 in Figure 55.3 ), along with remodeling (6 in Figure 55.3 ) of the scleral ECM. In tree shrews, and perhaps in mammals generally, these biochemical changes appear to control the biomechanical property of sclera, creep rate (6 in Figure 55.3 ). The creep rate is the extension of the sclera over time when a constant tension is applied. Normally in juvenile tree shrew eyes, scleral extensibility is low. As a result of the remodeling of the sclera, the creep rate rises. Loosely speaking, the sclera becomes more readily extensible. The changes in creep rate in turn allow normal intraocular pressure to increase the axial elongation rate of the eye (8 in Figure 55.3 ), moving the retina away from the cornea. In a hyperopic eye an increased axial elongation rate reduces the hyperopia, decreasing the defocus on the retina. Through this emmetropization feedback system, a hyperopic eye elongates until the visual signals (1 in Figure 55.3 ) that drive the feedback loop diminish and the eye stabilizes at emmetropia.
Scleral changes during myopia development and recovery
Many of the details of how the visual environment controls scleral remodeling summarized in the model shown in Figure 55.3 emanate from studies using form deprivation or negative lenses to induce the eyes to become myopic. Both interventions (“treatments”) produce a visual signal that stimulates the feedback loop, raising the amount of scleral remodeling, increasing the creep and axial elongation rates. Changes observed during recovery are generally the reverse of those found during myopia development.
When myopia is induced, the sclera becomes thinner, due in part to a reduction in the amount of type I collagen. Reductions of dry scleral weight of 3–5% develop after a few days of form deprivation or negative lens wear in tree shrews. Long-term form deprivation in tree shrew leads to a more substantial thinning, similar to that seen in humans with moderate myopia. Humans with pathological myopia have a marked scleral thinning, particularly at the posterior pole. In addition to scleral thinning, the collagen fibrils often show altered architecture in high human myopia, with a reduction in diameter, loss of longitudinal fibril striations, and a derangement of the growth and organization of the fibrils. Following long-term form deprivation in tree shrews, Norton and Rada noted a reduction in hydroxyproline (−11.8%) which suggests a loss of collagen. McBrien et al found similar changes: a reduction in median fibril diameter and misshapen collagen fibrils in tree shrews.
One advantage of animal models, in contrast to examination of human donor eyes, is that it is possible to examine the sclera and other tissues during the time an induced myopia is developing, rather than studying the postmortem endpoint of a longstanding myopic condition. After short-term form deprivation, although the sclera is thinner, the collagen fibrils appear normal in morphology, diameter, and spacing. During recovery, there is an increase in collagen synthesis and in mRNA expression level for type I collagen. These findings suggest that more subtle remodeling of scleral tissue occurs rather than destruction of structural elements, causing axial elongation and myopia development immediately after the onset of form deprivation or negative lens wear.
Animal model form deprivation study in relation to human myopia
Although animal models have provided information about how environmentally induced myopia can develop, questions have been raised about the applicability of animal studies to human myopia. For instance, form deprivation myopia has been reported to develop in young children with obstructive visual axis conditions such as ptosis or cataract. The myopic shift from form deprivation is inconsistent in humans, however.
An important similarity between animals and humans was noted by Gwiazda et al, who reported that progressing myopes (children with increasing myopia) underaccommodate to near visual targets more than do emmetropic children. The resulting hyperopic defocus resembles the hyperopic defocus that is produced by minus lens wear, which causes axial elongation in many animal species. This observation led to the “blur hypothesis,” which suggests that the eyes of children also elongate in response to the hyperopic defocus that occurs when the child underaccommodates to near targets. To the extent that accurate accommodation occurs, the defocus is reduced, which may be why the children with more accurate accommodation remained emmetropic in the Gwiazda et al study. The blur hypothesis is attractive as it not only relates animal studies to the human experience, but is also consistent with studies that have associated myopia development with near work. It contrasts with the suggestion, never supported by direct measurement, that accommodation itself is a cause of myopia by increasing tension on the sclera, stretching it so the eye elongates. The alternative provided by the blur hypothesis is that accommodation is beneficial, because it provides clear images on the retina and thereby removes the visual stimuli for increased axial elongation that can lead to myopia.
Human molecular genetics of myopia
Ocular refractive component genetics
The refractive state is determined by the relative contributions of the optical components, primarily corneal curvature, anterior-chamber depth, and lens thickness – all of which determine the location of the focal plane, and the axial length (primarily the vitreous chamber depth) – which determines whether the retina is located at the focal plane ( Box 55.3 ). Separately, these may be assessed as quantitative traits intimately related to the clinical phenotype of myopia. Multiple reports have examined familial aggregation and heritability of ocular components.
Molecular genetics studies of human myopia provide a relatively new translational possibility for determining genetic variants associated with myopia. The groundwork from these types of studies may enable the development of new therapies for treatment or prevention, or provide genetic tests for predicting those at greater risk for accompanying ocular morbidities of myopia
As expected from the previous sections, axial length is the largest contributor to the determination of refractive error. Several studies have reported an inverse relationship of axial length to refraction (the longer the eye, the more myopic the refractive error). Axial length of a myopic adult population may show a bimodal distribution with a second peak of increased axial length relating to high myopia (< −6 D at 24 mm, > −6 D at 30 mm) when plotted as a distribution curve. This suggests that myopia of −6 D or greater represents a deviation from the normal distribution of axial length and is not physiologic.
Estimates of heritability for axial length range from 40% to 94%. A study of three large Sardinian families found modest evidence for linkage on chromosome 2p24 with a likelihood of the odds (LOD) score of 2.64. Overall axial length includes anterior-chamber depth, and studies have shown that increased anterior-chamber depth has an inverse relationship as well to refractive error. The heritability reports for anterior-chamber depth range from 70% to 94%, and the same Sardinian study found modest linkage evidence to chromosome 1p32.2 with a LOD score of 2.32.
The steeper the corneal curvature, the more likely the resulting refractive error is myopic – eyes with hyperopia are more likely to have flatter corneal curvature readings by keratometry. Heritability estimates for corneal curvature range from 60% to 92%. The Sardinian family study noted modest linkage evidence of corneal curvature to chromosomes 2p25, 3p26, and 7q22 with LOD scores ranging from 2.34 to 2.50. Increased lens thickness correlates with increased myopia. A di- and monozygotic twin study reported 90–93% heritability for lens thickness.
Role of environment in myopic development
The prevalence of myopia in some populations appears to have increased dramatically from one generation to the next in progressively industrialized settings, or with increased level of educational achievement. Assessing the impact of inheritance on myopic development may be confounded by children adopting parental behavioral traits associated with myopia, such as higher-than-average near-work activities (i.e., reading). Observational studies of this risk factor do not fully explain the excessive familial clustering of myopia, however. A detailed assessment of confounding effects and interactions between hereditary and environmental influences in juvenile-onset myopia has shown that near work describes very little of the variance in refractive error compared to parental myopia. Additionally, near work exerted no confounding influence on the association between parent and child myopia, indicating that children do not become myopic by adopting parental reading habits. More importantly, there was no significant interaction between parental myopia and near work; reading was weakly and equally associated with myopia regardless of the number of myopic parents. This indicates that children could inherit myopia as a trait from parents. Of late, it has been proposed and accepted that relatively decreased outdoor activity, rather than near work, has a greater impact on myopic refractive error development.
Hereditary factors in myopic development
Multiple familial aggregation studies report a positive correlation between parental myopia and myopia in their children, indicating a hereditary factor in myopia susceptibility. Children with a family history of myopia had on average less hyperopia, deeper anterior chambers, and longer vitreous chambers even before becoming myopic. Yap and colleagues noted a prevalence of myopia in 7-year-old children of 7.3% when neither parent was myopic, 26.2% when one parent was myopic, and 45% when both parents were myopic. This implies a strong role for genetics in myopia.
Multiple familial studies support a high hereditary basis for myopia, especially higher degrees. Naiglin and colleagues performed segregation analysis on 32 French multiplex families with high myopia, and determined an autosomal-dominant (AD) mode of inheritance. The l s for myopia (the increase in risk to siblings of a person with a disease compared to the population prevalence) has been estimated to be approximately 4.9–19.8 for sibs for high myopia (−6.00 spherical D or greater), and approximately 1.5–3 for low or common myopia (approximately −1.00 to −3.00 spherical D), suggesting a definite genetic basis for high myopia, and a strong genetic basis for low myopia. A high degree of familial aggregation of refraction, particularly myopia, was reported in the Beaver Dam Eye Study population after accounting for the effects of age, sex, and education. Segregation analysis suggested the involvement of multiple genes, rather than a single major gene effect.
Twin studies provide the most compelling evidence that inheritance plays a significant role in myopia. Multiple studies note an increased concordance of refractive error as well as refractive components (axial length, corneal curvature, lens power) in monozygotic twins compared to dizygotic twins. Sorsby et al noted a correlation coefficient for myopia of 0 for control pairs, 0.5 for dizygotic twins, and almost 1.0 for monozygotic twins in a study of 78 pairs of monozygotic twins and 40 pairs of dizygotic twins. Twin studies estimate a notable high heritability value for myopia (the proportion of the total phenotypic variance that is attributed to genetic variance) of between 0.5 and 0.96.
Molecular genetic studies of human high-grade myopia
Several highly penetrant genetic loci for moderate and high myopia have been mapped (reviewed by Young et al). These loci are primarily AD or X-linked. They have been mapped in either large, isolated pedigrees, or populations derived from a limited number of founders that have experienced limited migration. Except for the X-linked MYP1 gene, none of the causative mutations has yet been found.
Much of the current information on human myopia molecular genetics can be drawn from studies of familial high-grade myopia, usually defined as spherical refractive error > – 5.00 D. An X-linked recessive form of myopia, named the Bornholm (Denmark) eye disease (BED), was designated the first myopia locus (MYP1: OMIM 310460) on chromosome Xq28. Collaborating with BED researchers, we made comparative molecular genetic haplotype and sequence analyses of a large Minnesota family of Danish descent that showed significant linkage of myopia to chromosome Xq27.3-q28. The phenotype of both families appears to be due to a novel cone dysfunction, and not simple myopia. The genetic etiology of each family appears to be distinct, as the haplotypes were different. A report by Michaelides et al confirms the different X-linked cone dysfunction syndrome with an associated high myopia phenotype as distinct from the BED in four families. The first identified myopia gene – CXorf2 at the MYP1 locus – has a distinct sequence mutation in the promoter region, and is a copy number variant.
Loci identified to date for isolated nonsyndromic high-grade myopia are primarily AD and highly penetrant. The first AD locus for nonsyndromic high-grade myopia was mapped within a 7.6-cm region on chromosome 18p11.31 (MYP2: OMIM 160700) in seven US families. This locus was confirmed in Chinese Hong Kong and Italian Sardinian cohorts. Using the Hong Kong cohort, investigators identified transforming growth-induced factor-β (TGIF-β) as the implicated gene for MYP1 using limited single-nucleotide polymorphism (SNP) association studies and exonic sequencing. Our group fully sequenced TGIF-β in our cohort of original MYP1 families, and found no associations with high myopia affected status. An Australian lab also investigated the association of TGIF with refraction and ocular biometric measurements in a Caucasian case-control population, and found no association. No association of TGIF to high-grade myopia case-control studies was found in Japanese and Chinese cohorts.
A second locus for AD high myopia mapped to a 30.1 cM region on chromosome 12q21-23 (MYP3: OMIM 603221) in an American family of German–Italian descent also in our laboratory. This locus was confirmed in a high-myopia Caucasian British cohort, and in a large German family. A statistically suggestive third locus for AD high myopia was reported on chromosome 7q36 in a Caucasian French cohort (MYP4: OMIM 608367). A fourth AD locus on chromosome 17q21-23 (MYP5: OMIM 608474) was determined in a large multigenerational English–Canadian family in our laboratory. We identified a locus for AD high-grade myopia on chromosome 2q37 (MYP12: OMIM 609994) in a large, multigenerational US Caucasian family. This locus was replicated in an Australian study of three multigenerational AD moderate myopia families. We determined a new locus (MYP17) on chromosome 10q21.2 in a Hutterite (Austrian/German) colony. Loci on chromosomes Xq23-25 (MYP13: OMIM 300613) and 4q22-27 (MYP11: OMIM 609994) were identified by Zhang et al in ethnic Chinese families. Other high-grade myopia loci have been recently mapped to chromosomes 15q12-13, 5p15.33-p15.2, and 21q23.3. The first locus implicated in ocular axial length, which is a major endophenotype for refractive error, was identified on chromosome 5q.
Juvenile-onset myopia genetics
At least two studies have shown nominal or no linkage of low to moderate myopia to many of the known high myopia loci. Mutti et al genotyped 53 common myopia families (at least one child with more myopia than −0.75D in each meridian) using the highest intrainterval LOD score microsatellite markers for the chromosome 18p and 12q loci and did not establish linkage. Ibay et al found no strong evidence of linkage to the chromosomes 18p, 12q, 17q, and 7q in a cohort of 38 Ashkenazi Jewish families with mild/moderate myopia (−1.00 D or more). These studies suggest that different genes account for mild/moderate myopia susceptibility or development, or that the gene effects are too small to be detected with the relatively small sample sizes. However, a recent study showed replication of the chromosome 2q37 (MYP12) locus in a cohort of Caucasian Australians with moderate to low myopia.
Three whole-genome mapping studies have identified several candidate gene intervals for common, juvenile-onset myopia using spherical refractive error data. The results of these studies demonstrate the potential for determining molecular genetic factors implicated in myopia at all levels of severity. These studies used microsatellite genotyping technology and a limited cohort sample size, and two used homogenous isolated populations. One study was a genome screen of 44 families of Ashkenazi Jewish descent. Individuals with at least −1.00 D of myopic spherical refractive error were classified as affected. Their strongest signal localized to chromosome 22q12 (MYP6: OMIM 608908) (heterozygous linkage of the odds = 3.56; nonparametric linkage = 4.62). Eight additional regions (14q, 4q22-q28, 8q22.2, 10q22, 11q23, 13q22, 14q32, and 17qter) had nominal linkage evidence. The same group replicated the same locus with additional Ashkenazi Jewish families. Hammond et al evaluated 221 dizygotic twin pairs with moderate myopia and found significant linkage to four loci, with a maximum LOD score of 6.1 on chromosome 11p13 (MYP7: OMIM 609256). Other identified loci mapped to chromosomes 3q26 (MYP8: OMIM 609257: LOD 3.7), 4q12 (MYP9: OMIM 609258: LOD 3.3), and 8p23 (MYP10: OMIM 609259: LOD 4.1). This group found that the PAX6 gene at the chromosome 11p13 locus showed linkage with 5 SNPs, but no association. They suggested that PAX6 (a major eye development gene) may play a role in myopia development, possibly due to genetic variation in an upstream promoter or regulator. This group later reported that neither of the master control genes PAX6 or SOX2 was implicated in common myopia in a large population study cohort. A report replicated the chromosome 8p23 myopia locus (MYP10) in an isolated Pennsylvania Amish population of 34 families. Other loci identified include chromosomes 1p36, 1q, 7p15, and 3q26.
Gene associations
High myopia also occurs as a feature of several ocular or systemic disease syndromes in which the causative disease gene is known ( Table 55.1 ). Candidate gene association studies have led to the identification of a number of high-myopia-susceptibility genes ( Table 55.2 ). These findings await replication in independent cohorts.
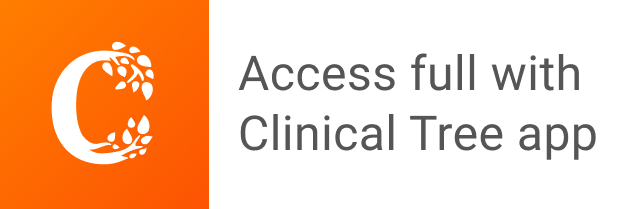