Fig. 4.1
Structure of the cochlea. A semi-thin plastic section through the cochlea of a 2-month-old rat. OC organ of Corti, TM tectorial membrane, SGN spiral ganglion neuron. Scale bar = 0.1 mm (Adapted from Ref. [119] with permission)
SGNs are anatomically and functionally divided into two subtypes, type I and type II. Type I SGN s occupy 90–95 % of all SGNs and have a large cell body, and their central neurites are thicker than peripheral neurites. The peripheral neurites of type I SGNs are ensheathed by peripheral-type myelin of Schwann cells. The central neurites are ensheathed by Schwann cells and by the central-type myelin of oligodendrocytes along their proximal (near the cell body of SGNs) and distal (near the brainstem) portion, respectively (Fig. 4.2). Type II SGN s comprise 5–10 % of all SGNs and have a smaller cell body. Their central and peripheral neurites are approximately the same thickness, and the peripheral neurites are unmyelinated [11–17]. Type I and II SGNs can be differentiated by their immunostaining pattern for the intermediate filament protein peripherin in mice and rats [18, 19]. The most important anatomical and functional difference between type I and II SGNs is the mode of connection of their peripheral neurites to hair cells (Fig. 4.3). Peripheral neurites of type I SGNs extend from the modiolus toward the organ of Corti in a radial fashion, and each of them makes synaptic contact with a single inner hair cell. Individual inner hair cells receive projections from 10 to 20 processes of type I SGNs. Peripheral neurites of the type II SGNs initially extend toward the organ of Corti in a similar manner as type I SGNs. After passing under the row of inner hair cells, each neurite changes direction in a spiral fashion parallel to an outer hair cell row and forms synapses on approximately three to ten outer hair cells [20–22]. Type I SGNs connecting to inner hair cells contribute to the transmission of sound information into the central auditory pathway, while type II SGNs that connect to outer hair cells are involved in active tuning of frequency in the cochlea [23–25].



Fig. 4.2
A transmission electron micrograph of SGNs from a 2-month-old rat. SGNs are ensheathed by Schwann cells. Scale bar = 5 μm

Fig. 4.3
Schematic drawing demonstrating the afferent neural connection in the cochlea. Peripheral neurites of type I SGNs extend from the modiolus toward the organ of Corti in a radial fashion, and each makes synaptic contact with a single inner hair cell. Individual inner hair cells receive multiple projections from type I SGNs. Peripheral neurites of type II SGNs initially extend toward the organ of Corti in a similar manner as type I SGNs. After passing under the row of IHCs, each neurite changes direction in a spiral fashion parallel to an OHC row and forms a synapse with an outer hair cell
The central neurites of type I and II SGNs pass through the internal auditory canal together with the superior vestibular, inferior vestibular, and facial nerves and reach the medulla, where they branch. The short anterior branches project to the ventral cochlear nucleus, and the long posterior branches reach to the dorsal cochlear nucleus [26, 27].
4.3 Development of SGNs
4.3.1 Neurogenesis
The first morphological sign of inner ear development is ectodermal thickening on both sides of the hindbrain, called the otic placode . The placode invaginates and pinches off the surface to become an epithelial vesicle called the otocyst . Otocyst formation occurs at 4 weeks of gestation in humans [28, 29] and embryonic 9.5 days in mice [30–32]. The otocyst undergoes complex shape changes, such as outward extrusion of the duct and epithelial fusion of the cyst wall, and then finally forms a membranous labyrinth consisting of three semicircular canals, the utricle, the saccule, the endolymphatic duct, and the cochlear duct [28–30, 33–36]. In mice, a cell group of future cochleovestibular ganglion can be differentiated at embryonic 10.5 days [37]. The origin of SGNs and whether they are derived from the otocyst or neural crest has long been controversial. An experimental study using chick-quail transplantation [38], as well as a very recent study to trace neural crest cells using a reporter gene [37], clarified that they are basically derived from the otocyst. On the other hand, a recent genetic fate-mapping study reports that SGNs have dual origin and are derived from the otocyst and neural crest [39].
The final cell division in the mouse spiral ganglion occurs at embryonic days 12–16 in mice [40]. After the final cell division, immature SGNs start differentiating and begin to extend central and peripheral neurites. Peripheral neurites penetrate into the organ of Corti at 9 weeks of gestation in humans [41] and embryonic day 11.5 in the mouse [42–44]. The projection of central axons into the cochlear nucleus is slightly later than the projection of peripheral axons into the organ of Corti [14].
4.3.2 Cell Death in SGNs
Studies in gerbils and rats have revealed that the number of SGNs in embryonic periods is approximately 20–30 % larger than that in adults. Neuronal number then decreases within a restricted postnatal time window (first postnatal week in both gerbils and rats) and becomes almost constant thereafter [45, 46]. Apoptosis of SGNs is upregulated corresponding to this period, suggesting that excessive SGNs are eliminated by neuronal cell death [47]. This regulation in neuronal number coincides with the postnatal refinement of cochlear afferent innervation [21, 22, 48]. The current hypothesis explains that SGNs are initially overproduced and that neurons compete with each other to contact their target (i.e., hair cells). The SGNs that have achieved the connection to hair cells are selected and survive, while SGNs that fail to establish a connection undergo apoptosis. Such a selection system is well known in the development of the nervous system to establish a mature innervation pattern [49, 50]. As described in Sect. 4.4, neurotrophic factors provided by hair cells appear to be deeply involved in this process.
4.3.3 Postnatal Rearrangement of Cochlear Afferent Innervation
Initial synaptic contact between hair cells and the peripheral neurites of SGNs in the embryonic period is subsequently remodeled to form their final neural circuit. Studies in gerbils and mice using axonal tracing and immunohistochemistry for type II SGN-specific peripherin have revealed that each afferent fiber of SGNs has contact with both inner and outer hair cells at birth. Most of the type I fibers then eliminate the contact with outer hair cells and retract to the inner hair cell region, while a small number of type II afferent fibers extend to form spiral fibers [21, 22]. The widespread arbors of type I fibers between inner hair cells undergo extensive pruning during this period, and each fiber finally has a synaptic contact with a single inner hair cell [21]. A study on rat SGNs suggested that a similar remodeling of afferent fibers begins around postnatal day (P)3–6 [48]. Interestingly, the expression of the brain-derived neurotrophic factor (BDNF) in the cochlear sensory epithelium, which disappears in the early postnatal period [48, 51], temporarily reappears at P6–P7 in hair and supporting cells [48]. In vitro studies have demonstrated that survival and neurite formation of SGNs in this developmental stage are supported predominantly by BDNF [52, 53]. Therefore, BDNF may play an important role in the rearrangement of innervation patterns of SGNs [48].
4.4 Neurotrophic Factors and SGNs
4.4.1 Expression of Neurotrophins and Their Receptors in the Cochlea
In many developing motor and sensory nervous systems, the establishment of neural circuits is strongly regulated by target-derived neurotrophic factors through the control of cell survival/death and neurite extension. The most well-characterized neurotrophic factors are members of the nerve growth factor (NGF) family of proteins [54, 55]. Four members of this family have been identified in mammals: nerve growth factor (NGF), BDNF, neurotrophin -3 (NT-3) , and neurotrophin-4/5 (NT-4/5). These molecules exert their effects on neurons through binding to their high-affinity receptors, members of the Trk family of receptor tyrosine kinases, TrkA, TrkB, and TrkC [56, 57]. The most well-known effect of neurotrophins is the enhancement of neuronal survival [54, 58–60], but a number of studies have elucidated multiple roles of these molecules on neuronal development, including the extension and morphogenesis of neurites [61–69].
In the developing auditory system, in situ hybridization and immunohistochemical studies revealed that BDNF and NT-3 are expressed in the organ of Corti, the target for SGNs [48, 51, 70–74]. At the same time, SGNs express their respective high-affinity neurotrophin receptors, TrkB and TrkC (Fig. 4.4) [59, 71–79].


Fig. 4.4
Expression of TrkB and TrkC in the rat cochlea. Paraffin sections from a 5-day-old rat cochlea were immunostained with rabbit polyclonal anti-TrkB (a) and anti-TrkC (b) antibodies. All SGNs express both TrkB and TrkC (Adapted from Ref. [120] with permission)
Detailed analysis of NT-3 and BDNF expression in mice along the apex-base axis has demonstrated that there is a gradient of expression within the cochlea for each neurotrophin during development [76]. In the early embryonic stage (embryonic day 12.5), BDNF expression is restricted to the apex of the cochlea, and expression spreads toward the basal cochlea later in development. In contrast, NT-3 expression is more confined to the middle and basal turn of the cochlea in the early stages of development, and the expression progresses toward the apex. Expression of both NT-3 and BDNF extends throughout the cochlea longitudinally by embryonic day 16.5 [76], implying that both neurotrophins are available to SGNs that have already come in contact with hair cells through their neurites. NT-3 is more strongly expressed than BDNF [51, 71, 76] and is distributed more widely in the sensory epithelium; both hair cells and supporting cells express NT-3, while BDNF is more restricted to hair cells during this stage [51, 76]. NT-3 continues to be expressed in inner hair cells and adjacent supporting cells of the postnatal and adult cochlea [80], while the expression of BDNF basically disappears in the cochlea [71, 72].
4.4.2 Neurotrophic Support for SGN Survival in Embryonic Development
Knockout mice null for the NT-3 or TrkC gene show considerably reduced numbers of SGNs at birth [81–83]. In contrast, BDNF or TrkB mutant mice mainly lose vestibular neurons [82, 83]. BDNF/NT-3 or TrkB/TrkC double homozygous mutants lose all cochlear and vestibular neurons around birth [83, 84].
More detailed analysis along the apex-base axis of the cochlea revealed that NT-3 knockout mice show an almost complete loss of innervation in the basal turns with a more mild decrease in innervation in the middle and apical turns [81]. In contrast, BDNF-deficient mice demonstrate mild loss of SGNs primarily in the apical turns [85]. These phenotypic differences in knockout mice would be explained by developmental changes in the expression of NT-3 and BDNF described above. When SGNs reach the critical period where they require neurotrophic support for survival, SGNs in NT-3 null mice are only supported by BDNF expressed in the apical region. Therefore, SGNs at the base of the cochlea die through apoptosis because of a lack of neurotrophic support. Conversely, in BDNF null mice, SGNs at this critical period are only supported by NT-3 expressed in the basal region, resulting in subsequent neuronal death in the apical region. The replacement of the NT-3-coding sequence with that of BDNF almost completely rescues the loss of basal turn SGNs by NT-3 absence [76, 86]. These results suggest that NT-3 and BDNF can be functionally equivalent for the survival of SGNs prenatally, and the specific pattern of neuronal loss in the cochlea of NT-3 and BDNF knockout mice is attributed to the availability of neurotrophic support during the critical period when SGNs need it for survival [76].
4.4.3 Other Neurotrophic Factors Involved in the Development of Cochlear Innervation
Recent studies have demonstrated that neurotrophic factors other than neurotrophins, such as glial cell-derived neurotrophic factor (GDNF) and ciliary neurotrophic factor (CNTF) , are also expressed in the auditory system. For example, GDNF is expressed in the postnatal cochlea, and the expression increases concomitant with hearing onset [87, 88], suggesting that GDNF may play a role in the adult function of the cochlea. GDNF promotes survival of SGNs both in vivo [87, 89] and in vitro [87, 90]. CNTF belongs to the interleukin-6 family of cytokines. The expression of CNTF is not only in the cochlea but also in the cochlear nucleus [88]. CNTF promotes SGN survival and neuritogenesis in vitro [91–93].
4.4.4 Neurotrophic Factors Promote Survival and Neuritogenesis of SGNs In Vitro
In vitro studies further support the hypothesis that both NT-3 and BDNF can promote SGN survival and neuritogenesis [59, 70, 73, 78, 79, 91, 94–98]. These studies have suggested that among the neurotrophic factors, BDNF shows the strongest promoting effects of survival and neurite formation on early postnatal SGNs (Fig. 4.5).


Fig. 4.5
The effect of BDNF on neuritogenesis in 5-day-old rat SGN explants. The explants were cultured for 3 days in media either without neurotrophin (a) or supplemented with 10 ng/ml of BDNF (b) and then fixed and immunostained with anti-NF200 antibody. The number of neurites sprouting and the length of neurite extension were significantly increased following supplementation with BDNF. Scale bar = 0.5 mm (Adapted from Ref. [119] with permission)
We recently examined the age-dependent changes in responsiveness of SGNs to NT-3, BDNF, and leukemia inhibitory factor (LIF) using dissociated cultures [53]. Our data suggest that these neurotrophic factors may predominantly support different ontogenetic events in different developmental stages in the innervation of the inner ear (Figs. 4.6 and 4.7).



Fig. 4.6
(a) An example of cultured SGNs after 12 h in primary growth media containing serum and a further 72 h in serum-free maintenance media. Cells were fixed and immunostained with anti-NF200 antibody. Surviving SGNs were identified as NF200-positive cells. Cultured neurons either had no neurites (indicated as N) or were monopolar (indicated as Mo; with one neurite emanating from the cell body), bipolar (indicated as B; with two neurites emanating from the cell body), or multipolar (indicated as Mu; with three or more neurites emanating from the cell body) morphologies. High-magnification views of N, Mo, B, and Mu SGNs shown in (a) are in (b), (c), (d), and (e), respectively. Scale bars = 0.5 mm in (a) and 50 μm in (b–e) (Adapted from Ref. [53] with permission)

Fig. 4.7
The effects of neurotrophic factors on survival and neuritogenesis in 5-day-old rat SGNs in dissociated cultures. Neurons were cultured for 12 h in serum-containing primary growth media and for a further 72 h in serum-free maintenance media without any neurotrophic factors (a; C), or supplemented with 50 ng/ml of NT-3 (b; N), BDNF (c; B), LIF (d; L), a combination of NT-3 and BDNF (50 ng/ml each; N+B) (e), or a combination of NT-3, BDNF, and LIF (50 ng/ml each; ALL) (f) and then fixed and immunostained with anti-NF200 antibody. Survival effects of NT-3, BDNF, LIF, and their combinations compared with untreated control are shown. The length of neurites appeared to increase following treatment with LIF or ALL factors (arrowheads in d, f). Scale bar = 0.5 mm (Adapted from Ref. [53] with permission)
In this analysis, we demonstrated that LIF has a strong effect on neurite extension of postnatal SGNs (Fig. 4.7). LIF is a member of the interleukin-6 family as CNTF and has been reported to enhance neurite extension in a variety of other neuronal types [99, 100]. In particular, recent studies have demonstrated that LIF mediates the enhanced intrinsic growth status after a conditioning lesion [99, 101], suggesting that LIF can play a role in the regeneration of injured neurites. Therefore, our results, together with those in previous reports [92, 93, 97], suggest that the application of exogenous LIF, alone or together with neurotrophins, may be clinically valuable as a treatment for central axon injury from trauma or surgical removal of acoustic tumors, as well as for peripheral dendrites to improve the efficacy of cochlear implants.
4.4.5 Damage of the Organ of Corti and Secondary Degeneration of SGNs
In the adult inner ear, hair cells (especially inner hair cells) appear to be the major source of neurotrophic support to SGNs. The loss of hair cells induces secondary loss of SGNs presumably because of a loss in neurotrophic support [88]. In such secondary degenerative processes, the peripheral neurites first retract following hair cell loss, and the degeneration of SGNs occurs in long-term deafness [102]. The speed of degeneration appears to depend on the species; in deafened rats and guinea pigs, SGN death occurs over a few months [103–106], whereas in deafened cats, SGN death occurs over months to years [107]. In humans, SGNs are capable of surviving for many years in the absence of hair cells [108, 109]. The reason underlying this interspecies difference is unclear.
4.4.6 Therapeutic Potential of Neurotrophic Factors in Cochlear Implant Therapy
The degenerative process of SGNs can deteriorate the efficacy of cochlear implants. Therefore, the development of a therapeutic strategy to prevent the degeneration of SGNs is needed. When the deafened ear is treated with exogenous BDNF, NT-3, or other neurotrophic factors, there is a significant enhancement of SGN survival [110–116]. Therefore, chronic application of NTs in the cochlea may have benefits in maintaining the efficacy of cochlear implants. It is interesting that despite the limited endogenous expression of BDNF in the mature adult cochlea, the SGNs retain the capacity to respond to BDNF for survival. This finding agrees with that of our in vitro study, in which rat SGNs harvested from postnatal day 20, when almost all major developmental events for SGNs are complete and hearing function has matured by this age [16], respond to BDNF for survival [53].
Such exogenous neurotrophic factors not only prevent the cell death of deafferentated SGNs but also promote the regrowth of peripheral processes toward a new source of neurotrophic factors that act as chemoattractants [110, 113, 114, 117]. Resprouting of the peripheral neurites of SGNs would enable the reduction of stimulation current, thereby diminishing current spread and improving the number of perceptual channels achieved with cochlear implant electrode arrays. It may also enable the use of CI electrodes that have a larger number of channels.
To deliver neurotrophic factors into the cochlea, intracochlear administration using osmotic pumps and viral vectors has been used to provide trophic factors to the cochlea (for review, see [3, 5]). We recently developed a CI electrode array coated with a biocompatible 2-methacryloyloxyethyl phosphorylcholine (MPC) polymer [118], which is expected to ensure safe and reliable insertion and anti-inflammatory effects. MPC polymers contain extremely hydrophilic phosphorylcholine in their side chains, and surfaces covered with an ultrathin (50 nm) MPC layer have been shown to exhibit good wettability and low friction. MPC polymers have been applied to several medical devices, such as artificial hip joints, implantable blood pumps, cardiovascular stents, oxygenator, and soft contact lenses. One of the potential advantages of using MPC polymer to coat cochlear implant electrodes is that MPC polymer can be a reservoir for various factors, including neurotrophic factors. There is great hope that in the near future, modified MPC polymers that elute such substances will play a key role in enhancing performance after CI.
References
1.
Yang T, Kersigo J, Jahan I, Pan N, Fritzsch B. The molecular basis of making spiral ganglion neurons and connecting them to hair cells of the organ of Corti. Hear Res. 2011;278:21–33. doi:10.1016/j.heares.2011.03.002.PubMedPubMedCentral
2.
Delacroix L, Malgrange B. Cochlear afferent innervation development. Hear Res. 2015;330:157–69. doi:10.1016/j.heares.2015.07.015.PubMed
3.
Ramekers D, Versnel H, Grolman W, Klis SF. Neurotrophins and their role in the cochlea. Hear Res. 2012;288:19–33. doi:10.1016/j.heares.2012.03.002.PubMed
4.
Green SH, Bailey E, Wang Q, Davis RL. The Trk A, B, C’s of neurotrophins in the cochlea. Anat Rec (Hoboken). 2012;295:1877–95. doi:10.1002/ar.22587.
5.
Budenz CL, Pfingst BE, Raphael Y. The use of neurotrophin therapy in the inner ear to augment cochlear implantation outcomes. Anat Rec (Hoboken). 2012;295:1896–908. doi:10.1002/ar.22586.
6.
Spoendlin H, Schrott A. Quantitative evaluation of the human cochlear nerve. Acta Otolaryngol Suppl. 1990;470:61–70.PubMed
7.
Ishiyama G, Geiger C, Lopez IA, Ishiyama A. Spiral and vestibular ganglion estimates in archival temporal bones obtained by design based stereology and Abercrombie methods. J Neurosci Methods. 2011;196:76–80. doi:10.1016/j.jneumeth.2011.01.001.PubMed
8.
Tang Y, Lopez I, Ishiyama A. Application of unbiased stereology on archival human temporal bone. Laryngoscope. 2002;112:526–33. doi:10.1097/00005537-200203000-00022.PubMed
9.
Hall RD, Massengill JL. The number of primary auditory afferents in the rat. Hear Res. 1997;103:75–84. doi:10.1016/S0378-5955(96)00166-9.PubMed
10.
Keithley EM, Feldman ML. Spiral ganglion cell counts in an age-graded series of rat cochleas. J Comp Neurol. 1979;188:429–42. doi:10.1002/cne.901880306.PubMed
11.
Perkins RE, Morest DK. A study of cochlear innervation patterns in cats and rats with the Golgi method and Nomarkski Optics. J Comp Neurol. 1975;163:129–58. doi:10.1002/cne.901630202.PubMed
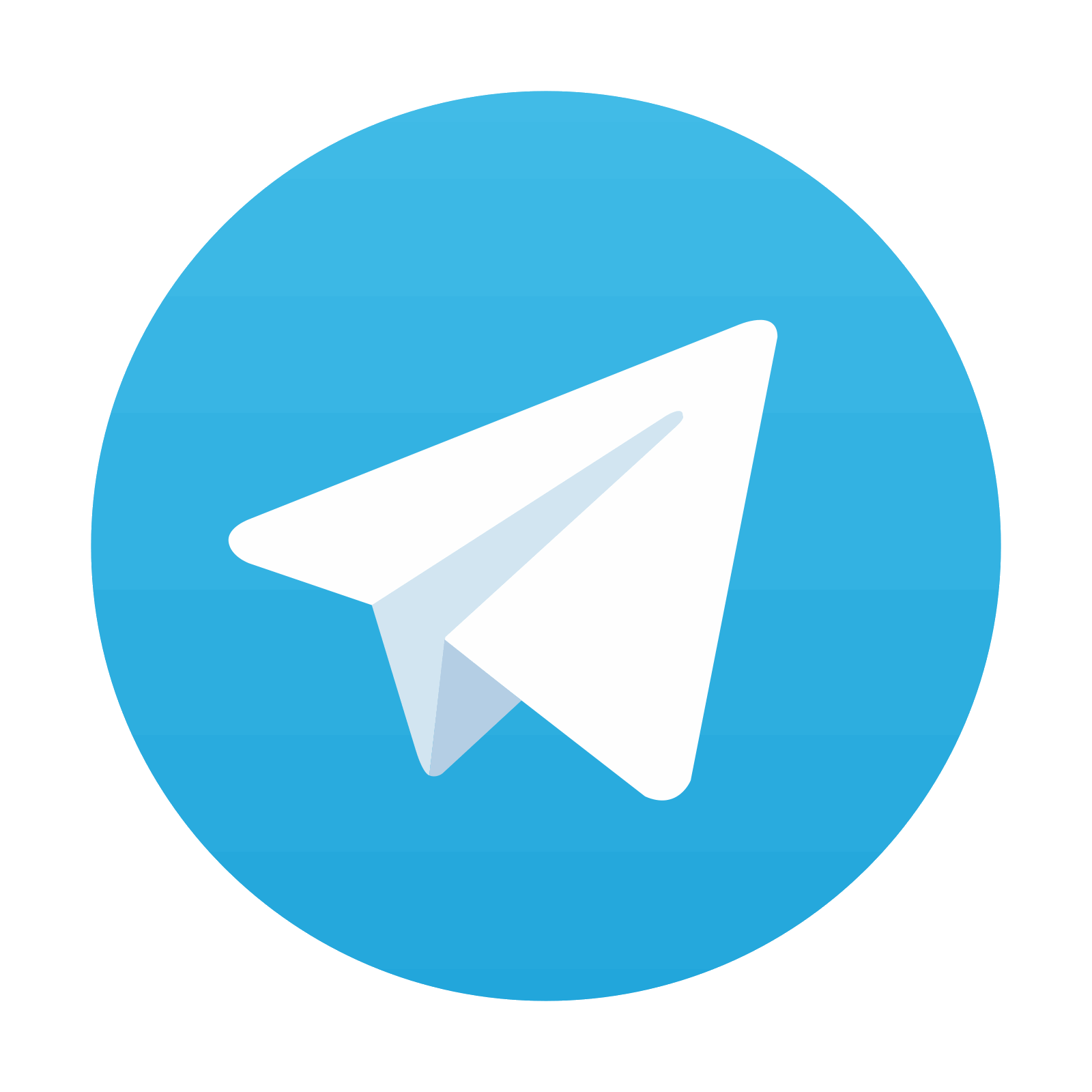
Stay updated, free articles. Join our Telegram channel
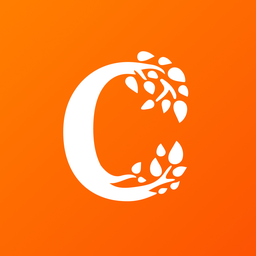
Full access? Get Clinical Tree
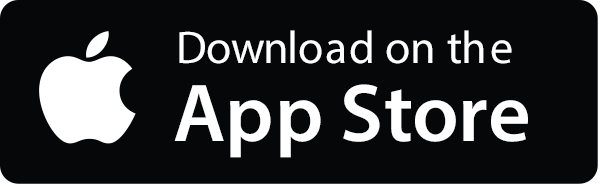
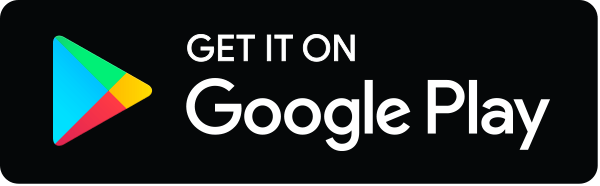