Fig. 24.1
(a) 5 kHz tone stimulus (top) and neurophonic response (bottom). (b) 5 ms interval of the neurophonic at tone onset (Modified from Kuokkanen et al. 2010)
3.1 Dorsoventral Maps of ITD in Owls Derived from Tone-Induced Responses
Within NL, binaurally evoked neurophonics vary with interaural phase difference (IPD), as do ITD-sensitive responses recorded from NL neurons (Carr and Konishi 1990; Peña et al. 2001). The ITD that evokes the maximum neurophonic response changes systematically, always shifting towards ipsilateral space with increasing depth (Sullivan and Konishi 1986); Fig 24.2a). These location-dependent shifts in best ITD occur only within NL; lesions marking the onset of the systematic shift are found on the dorsal edge of the nucleus, and those marking the end of the phase shift are on the ventral edge (Sullivan and Konishi 1986).
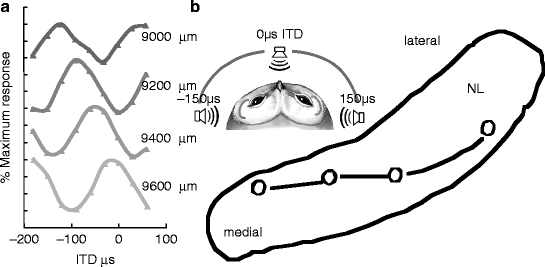
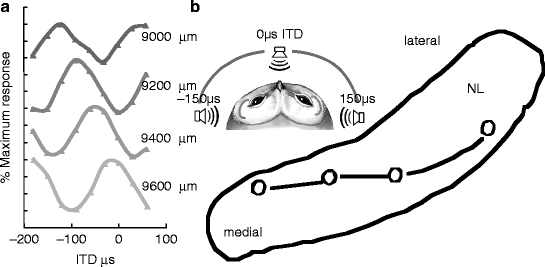
Fig. 24.2
(a) Neurophonic responses recorded at 200 μm intervals in a single penetration through NL. (b) Reconstructed section through NL, showing location of lesions placed at 0 μs ITDs in multiple penetrations and revealing that iso-ITD lines were not parallel to the borders of NL. Inset: schematic owl head showing range of ITDs (von Campenhausen and Wagner 2006)
We measured the changes in the neurophonic obtained when traversing the short, dorsoventral axis of NL. From dorsal to ventral within NL, the phase delay of a contralaterally elicited potential decreased and that of its ipsilateral counterpart increased (Sullivan and Konishi 1986). This neurophonic delay reflects the delay of phase-locked spikes originating from interdigitating ipsi- and contralateral axons from NM and generates an orderly representation of delay disparities.
NL is tonotopically organized, and its isofrequency laminae are oriented along a plane that is inclined about 45° to the midline. We quantified the distribution of ITDs within a single isofrequency lamina by stereotactically sampling ITD at multiple locations within this plane. Each isofrequency lamina contained a map of optimal binaural delays. The map is best illustrated by iso-delay contours (Fig. 24.2b).
Medial portions of each tonotopic lamina mapped best IPDs and ITDs near 0 μs dorsally in NL, with a representation of ipsilateral space below (Fig. 24.2b). The central portions of the tonotopic lamina contained maps of best ITD that were centered on frontal space. The most lateral lesions were characterized by representations of contralateral space, and the most extreme lateral positions did not include a representation of 0 μs. Thus, there was a steady shift in the mapping of 0 μs from dorsal in medial NL to ventral in lateral NL.
3.2 ITD at 0 μs Can Be Derived from Latencies of Click-Induced Responses
Since the representation of frontal space shifted systematically to more ventral locations with mediolateral progression within each tonotopic band, we measured delay latency at different mediolateral positions to determine the basis of the shift and to show how regulation of conduction velocity could underlie map formation. Click stimuli provide a useful measure of conduction delay, since click phase is precise (Wagner et al. 2005). We compared the neurophonic responses to ipsi- and contralateral clicks at best ITDs at or near 0 μs and observed overlaid, phase-locked but not identical ipsi- and contralateral click responses (Fig. 24.3a).
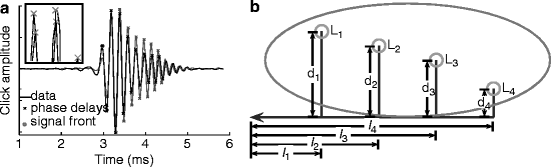
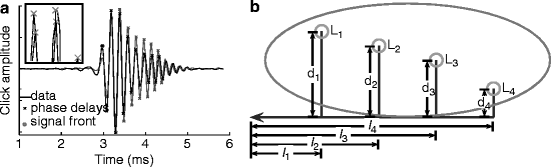
Fig. 24.3
(a) Left and right neurophonic click responses recorded at 0 μs best ITD. (b) Model of neurophonic conduction velocity accounts for observed shifts in best ITD. Mediolateral lengths are marked l1-4, while dorsoventral lengths are marked d1-4
3.3 Models of Conduction Velocity and Maps of ITD
We developed a simple linear model of NL that incorporated click delays (Fig. 24.3b). Conduction velocities along the delay lines inside (d1–d4) and outside NL (L1–L4) were assumed to be different but constant, consistent with our measures of axon diameter and internodal distance (Carr and Konishi 1990; Carr and Boudreau 1993). Also, we assumed a common path from NM to the midline, followed by a measured path from the midline to the edges of NL. Axon lengths were estimated using a predefined straight-line geometry, derived from reconstructions of axon paths. The parameters of this geometry were fit to three-dimensional reconstructions of the NL borders and the locations of lesions reconstructed from counterstained sections.
The model was used to evaluate possible conduction velocities along the delay lines in NL. The model yielded a range of possible conduction velocities lying between 1 and 10 m/s with velocities within the nucleus being smaller than those outside NL. The model also showed that iso-ITD lines cannot run parallel to the dorsal and ventral borders of NL in the mediolateral direction (Sullivan and Konishi 1986), because parallel iso-ITD lines yielded biologically unrealistic conduction velocities, which is consistent with our experimentally observed iso-ITD contours.
3.4 Plasticity in Maps of ITD
Young owls (from P21) were unilaterally fitted with an ear-canal insert designed to introduce a time delay to inputs from that ear (Gold and Knudsen 1999). After growing up with this altered auditory experience, the representation of ITD in NL was mapped using neurophonic responses and tested for any differences to normal. Our hypothesis was that if NL adjusts for the temporally altered input, the maps of ITD would be shifted compared to normal. In addition, complementary shifts are expected in the NL of both sides. Specifically, on the side ipsilateral to the ear insert, the point of zero ITD should shift ventrally and dorsally on the contralateral side. Preliminary analysis did not reveal any shifts in the predicted, adaptive direction. This suggests that nucleus laminaris does not show experience-dependent plasticity, at least not after P20–30. The data are consistent with the observation that myelination of the delay lines peaks at the third week posthatch (Cheng and Carr 2007).
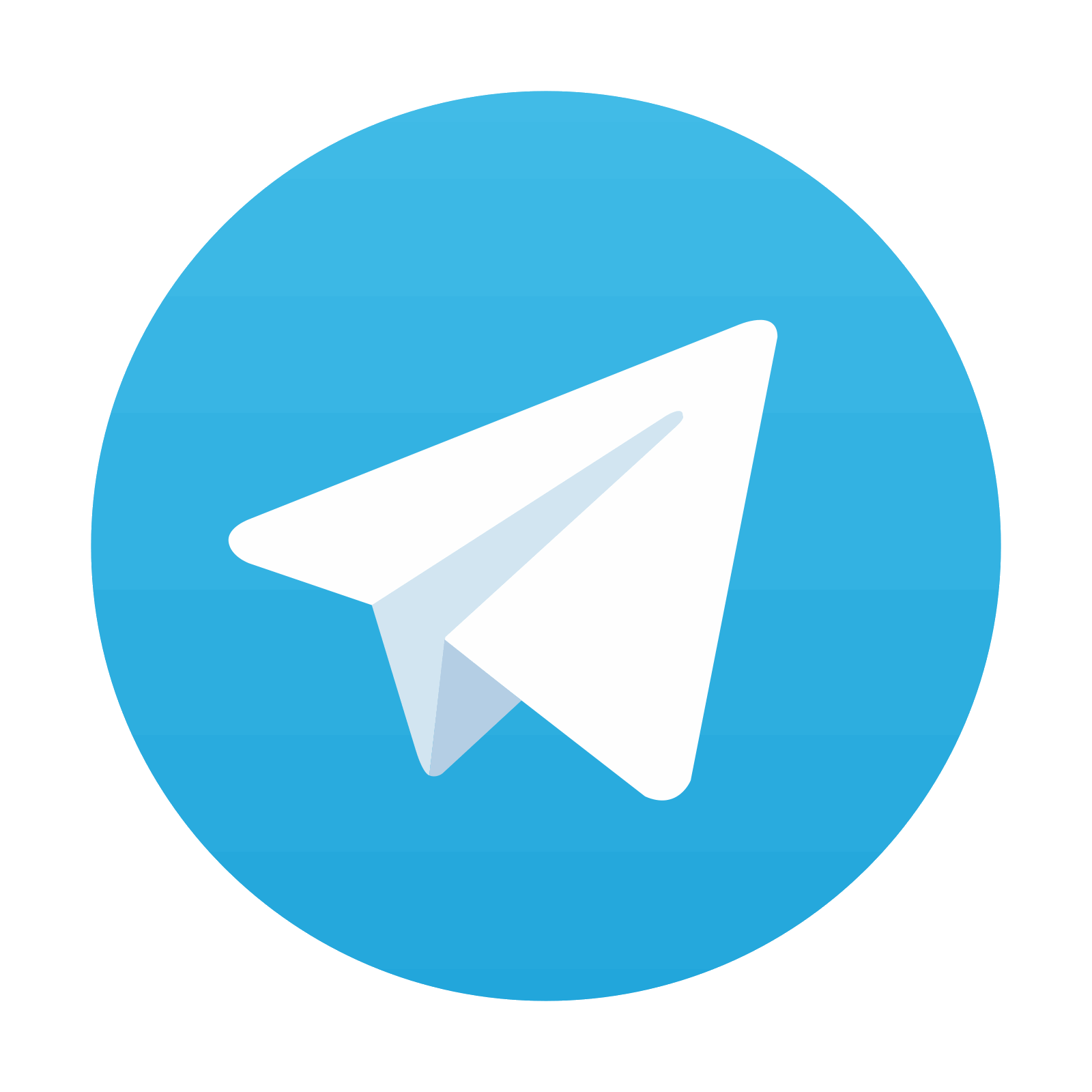
Stay updated, free articles. Join our Telegram channel
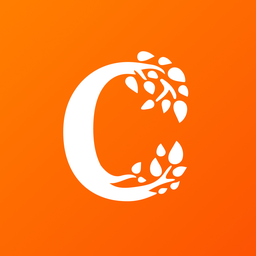
Full access? Get Clinical Tree
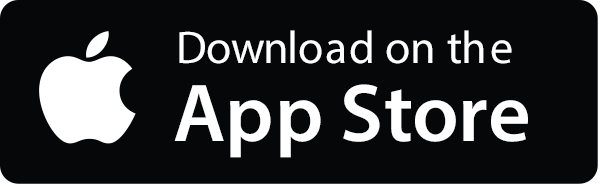
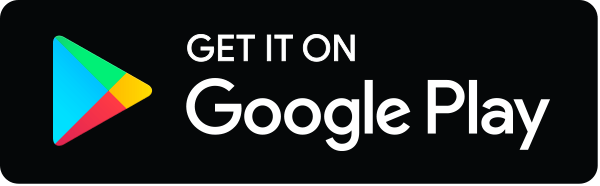