Lens
Norman J. Kleiman
Basil V. Worgul
The present understanding of lens anatomy can be traced to the very beginnings of microscopy. The lens has the distinction of being among the first tissues to be examined microscopically; van Leeuwenhoek himself described the fiber organization of lenses from cow,1,2 ox,1,2 hare, rabbit, cod, bird,2 and, most significant, human3,4 eyes. Despite those pioneering observations, however, subsequent studies concentrated, for the most part, on cataracts and cataract surgery. Nevertheless, by the second half of the nineteenth century, numerous lens studies had been reported,5 which led to renewed interest in lens microanatomy.* These early investigations have set the stage for present-day studies of lens biology and pathology.
The lens is not simply a “bag of protein,” as one popular reductionist metaphor opined, but is instead a highly organized cytosystem exquisitely designed to carry out its sole function (i.e., to refract incoming light upon the retina). This singular role necessitated the evolution of unique anatomic characteristics, an appreciation of which can be best acquired by first understanding the nuances of the embryogenesis of the lens.
EMBRYOLOGY OF THE LENS
Morphologically, the development of the human lens begins at the 4-mm embryonic stage (Table 1). A section through the area of the forebrain (Fig. 1) reveals that lateral outpocketings in the totally internalized neural ectoderm, called the optic vesicles, come in close apposition to the surface ectoderm. The union of these tissues initiates the formation of the lens placode (Fig. 2), an area of columnarization or “palisading”6 of the monolayered surface ectoderm in the region adjoining the neural ectoderm. This induction is the first of a series that governs the formation and growth of the lens.7,8 The precise nature of the inductive agent(s) is unknown, but it is believed that a multistep process occurs that does not require direct contact between cell types.9,10,11,12,13 Although the process is still not well understood, it is likely that a number of inducing agents and/or growth factors are involved.14,15,16,17,18,19
TABLE ONE. Important Stages in the Development of the Human Lens | ||||||||||||||||||||||||||||||||||||||||||||||||
---|---|---|---|---|---|---|---|---|---|---|---|---|---|---|---|---|---|---|---|---|---|---|---|---|---|---|---|---|---|---|---|---|---|---|---|---|---|---|---|---|---|---|---|---|---|---|---|---|
|
Although the lens placode appears stratified, it remains a single layer of columnar cells. The apparent multilayering is caused by the staggered position of nuclei in adjacent cells. The variable location of nuclei along the length of their respective cells may be due to a cell cycle-dependent migration of the nuclei of the lens placode cells. The work of Zwaan and his associates has shown that, following deoxyribonucleic acid (DNA) synthesis, the nuclei located basally in the lens placode migrate to the cell apices prior to mitosis.20 Following cell division, the nuclei of the resulting daughter cells return to the basal location.
A small depression called the fovea lentis (lens pit) appears below the center in the lens placode (see Fig. 2). This will be the focus of the invagination of the surface ectoderm that forms the lens vesicle. As the crowding of the lens placode cells increases, the lens pit begins to deepen (Fig. 3). Because the lens pit was initially off-center, invagination of the surface ectoderm occurs asymmetrically. Concurrently, the optic vesicle invaginates as it undergoes conversion into the double-layered optic cup. Flat cells appear on the outer surface of the lens plate, predominantly in the area of the lens pit (see Fig. 3). At this stage (5 mm), patches of similar cells may be found elsewhere over the embryo. These epitrichial cells are the beginning (periderm) of the simple squamous epithelium that is destined to cover the entire embryo. Although they first accumulate in the concavity of the lens pit, the epitrichial cells begin to disappear by the 7-mm embryonic stage (4 weeks). Pari passu with their elimination, the lens pit deepens considerably, and the opening to the surface ectoderm, the lens stalk, constricts to the point where the lens pit may now be referred to as the lens vesicle. Following complete closure, the vesicle begins to separate from the presumptive cornea at approximately the 9-mm stage (Fig. 4). Phagocytic macrophages, whose role is to remove dead cells and debris, have been detected in the interspace between the vesicle and ectoderm as well as in the lens lumen.21 At this time, the vesicle is spherical and approximately 0.2 mm in diameter. Its wall is about 40 μm thick.
Because the vesicle represents an invagination of surface ectoderm, the basal portions of its cells are oriented externally, whereas the apices of the cells face the lumen. Because of this relationship, components of the basal lamina (secreted by the lens cells throughout life) are deposited externally and encase the tissue in a membranous envelope, later to be called the lens capsule. Electron microscopy reveals that capsule formation begins at about the 10-mm stage,22 just after the vesicle and surface separate. Capsule formation is initiated by the appearance of a second basal lamina deposited in a discontinuous manner23 beneath the original basement membrane of the lens vesicle cells. (The readily discernible periodic acid-Schiff (PAS) stain positive structure familiar to the light microscopist will not appear until approximately the 13-mm stage.)
Concomitant with the beginnings of capsule formation is the appearance of the first vessels of the tunica vasculosa lentis (Fig. 5), a vascular net that will completely encompass the lens by the 40-mm stage. It begins as small capillaries emanating from the hyaloid artery and covering only the posterior portion of the lens (posterior vascular capsule). From the posterior vascular capsule, palisade vessels, visible at the 12-mm stage, proceed anteriorly around the equator where they anastomose with choroidal veins (capsulopupillary portion). From these arise the vessels that will cover the anterior lens surface, in conjunction with branches of the long ciliary arteries, to form the so-called pupillary membrane (anterior vascular capsule). Fully developed by the 40-mm stage, the vascular capsule will completely disappear before or soon after birth. Phagocytic macrophages have been implicated in its destruction.24 (Not infrequently, however, a hyaloid remnant or a small portion of the pupillary membrane persists in otherwise normal eyes.
Inside the lens, the 10-mm stage also heralds a number of differentiating activities. At the biochemical level, expression and synthesis of various lens structural proteins, the crystallins25 can be detected.26,27,28,29,30 In the mammalian lens, the crystallins are divided into three groups, termed α, ß, and Γ on the basis of their chromatographic elution profiles, acid sequence, and immunologic cross-reactivities. Typically, α crystallin transcripts are expressed first, followed closely by ß crystallin. In contrast, Γ crystallin expression and synthesis is closely linked to the later elongation phase of the terminally differentiating fiber cell.
Morphologically, the posteriorly situated cells of the vesicle (i.e., those nearest the presumptive retina) begin to elongate (see Fig. 4). As they do, they encroach upon the lumen. Because those cells that are located at the posterior pole elongate first, they advance ahead of the more equatorially placed cells. As a result, the cavity of the lens vesicle becomes increasingly more crescentic and smaller (Fig. 6). The elongation of the posteriorly placed lens vesicle cells represents another step in the string of inductions controlling lens formation and development. In a classic series of experiments, Coulombre and Coulombre observed that if the lens vesicle is rotated 180° during the elongation process, the induction pattern can be reversed: the elongation of the cells, which are now located anteriorly, terminates, whereas the original anterior cells, those now nearest the retina, lengthen.31 This interaction between retina and lens tissue persists into adulthood.32,33,34,35
The posteriorly located vesicle cells elongate until, at approximately the 16-mm embryonic stage, the lumen is completely obliterated. The nuclei of these transformed epithelial cells (now referred to as the primary fibers of the lens) assume a position more anterior than posterior along the length of the fibers. At this stage, the cells of the anterior portion of the original lens vesicle remain morphologically unchanged. Hereafter, this monolayer of cells will be referred to as the lens epithelium, and upon it all future growth of the lens depends. Except for the progressive denucleation of the innermost cells of the primary fiber mass, and the reversion of the lens epithelium to squat cuboidal cells (Fig. 7), little change occurs in the lens as the embryo grows from the 16-mm to the 25-mm stage. At the 25-mm stage, the lens epithelium displays a tremendous burst of proliferative activity, and the epithelial cells in the area of the equator begin to differentiate into fiber cells (Fig. 8). It is believed that a variety of growth factors,15 including the insulinlike growth factors34 and fibroblast growth factor,16 as well as various cellular proto-oncogenes,36,37 play essential roles in this process. The change in wet weight of the embryonic human lens from 8 to 12 weeks’ gestation is shown in Figure 9.
Following terminal cell division in the preequatorial area, just anterior to the lens equator, lens epithelial cells move into the equatorial region, where they rotate 90° and elongate (see Fig. 8). As they lengthen, the anterior ends pass in front of, and the posterior ends behind, the primary fiber cell mass, thereby completely surrounding and internalizing it. As new fibers are added around the primary fibers (secondary fibergenesis), each new layer grows longer than its predecessor. A secondary fiber cell, however, is never long enough to reach both the anterior and posterior poles of the lens. Instead of converging at a single point, within each layer or growth shell, the cell termini abut in a manner producing visual patterns known as lens sutures.38
The first recognizable suture is Y-shaped (at about the 35-mm stage) and is present in layers laid down from early embryogenesis to birth (Fig. 10A). Lens suture patterns become increasingly more complex with each succeeding growth shell. For example, young adults typically have nine suture lines arrayed in a starburst pattern (Fig. 10B), whereas older individuals have a more complex and multibranched sutural arrangement (Fig. 10C).39,40,41
As the lens increases in size, it becomes apparent that each layer of fiber cells will overlap equatorially but not necessarily in the anterior and posterior regions. This results in the equatorial diameter becoming significantly greater than the diameter along the anteroposterior axis (Fig. 11). Functionally, this manner of fiber cell deposition provides for the transformation of the lens from a spherical shape during embryogenesis to a more refractively favorable biconvex lentoid shape in the adult eye.
THE ADULT LENS
The internal anatomy of the adult human lens is shown in Figure 12. From birth, the lens undergoes little qualitative change, although quantitatively its volume, weight, and size continue to increase throughout life (Table 2). Male lenses are heavier than female lenses of patients the same age,42 although the rate of increase in weight is approximately the same. The lens becomes markedly ellipsoid during the early portion of postnatal life, but after the 20th year the rate of anteroposterior growth exceeds the rate of equatorial growth, thus causing the lens to become rounder with increasing age.43,44,45,46 The length of the anteroposterior axis increases at a rate of about 0.023 mm/year throughout life44,45,47,48 (Fig. 13), whereas the equatorial axis increases from about 6 to 9 mm during the first 15 years of life, changing little thereafter. A large variation is observed in the radius of curvature of the anterior and posterior surfaces of the adult lens. The average radius of curvature of the anterior surface is approximately 10 mm (8–14 mm), and that of the posterior surface is about 6 mm (4.2–7.5 mm).45,49,50 The radius of curvature becomes increasingly smaller with age (Fig. 14).44,45,46
TABLE TWO. The Change in Wet Weight, Volume, and Front Diameter of the Lens with Age | ||||||||||||||||||||||||||||||||||||||||||||||||
---|---|---|---|---|---|---|---|---|---|---|---|---|---|---|---|---|---|---|---|---|---|---|---|---|---|---|---|---|---|---|---|---|---|---|---|---|---|---|---|---|---|---|---|---|---|---|---|---|
|
THE LENS EPITHELIUM
Like the skin (its embryonic sibling), the lens grows throughout life, albeit at a rate that decreases with age. From the start of secondary fiber formation at the 25-mm embryonic stage, all lens growth becomes dependent on the mitotic activity of the lens epithelium.
The epithelium, a polarized monolayer of cells occupying the anterior surface of the adult lens, is derived from the original lens vesicle cells that did not differentiate into primary fibers. Estimates of cell number range from 350,00051 to 100,000,00052 in the mature human lens. The epithelium does not extend to the posterior side of the lens because the cells originally located there have elongated into primary fibers. Because it adheres tenaciously to the overlying capsule, it is possible to remove the entire population of epithelial cells. Once isolated, the intact epithelium can be mounted on a slide and subjected to qualitative and quantitative histocytologic analyses. Techniques such as autoradiography53 and histochemistry of lens epithelial whole-mount preparations have revealed that epithelium is organized into several distinct subpopulations, distinguished primarily by their proliferative kinetics,54,55 although there are some morphologic differences among them.
The cells located centrally on such preparations (i.e., those that would occupy the anterior pole of the lens in sagittal section) are polygonal with round nuclei when viewed on a whole mount and squamous with elliptically shaped nuclei when viewed in section (Fig. 15). They are flat, with a considerable range of cell diameters, an average height of approximately 6 μm, and a width of 13 μm,56 and they are endowed with all of the organelles typical of epithelial cells. Cells located more posteriorly tend to be smaller, and, consequently, cell density increases toward the equator.51 In the adult male, the age-adjusted mean cell density is about 5000 cells/mm2, and it is about 5800 cells/mm2 in females.57 The average cell density declines with age. In epithelium from cataractous lenses, both values are lower.58,59
Lens epithelial cells contain defined apical, lateral, and basal membranes; their apical surface interfaces with the apical membranes of newly formed fiber cells.60 Considerable endocytosis occurs, presumably reflecting transport of nutrients between epithelial and fiber cells.61 It is likely that no tight junctions62 and very few gap junctions are found in this region. In contrast, at the lateral membranes, characterized by complex interdigitations, are found desmosomes (macula adherens) and plentiful numbers of gap junctions.63
The central zone cells do not normally undergo mitosis. In fact, the mitotic index for cataractous epithelia from aged individuals has been reported to be as low as 0.0004%,64 although values as high as 0.008% for both normal and cataractous lenses have been reported.51 However, experimental animal studies have shown that the central zone cells can respond to myriad mitotic stimuli, which include injury,54,55,65,66,67,68,69,70,71,72 explantation,54,55,73 hormones,74,75,76 and experimental uveitis.77,78,79,80 Because they are relatively easy to manipulate, these normally noncycling cells constitute a model system of particular interest to investigators studying the processes of wound healing and the control of the cell cycle.54,55 The cells of the anterior pole have also gained the attention of gerontologists, a fact that is not surprising because it is believed that these cells remain centrally located without migrating out of the region. They are, as Muggleton-Harris states, “a true collection of aged cells.”80
Biochemically, lens epithelial cells are relatively unremarkable. A variety of cytoskeletal proteins and components, including actin, vimentin, myosin, microtubules, spectrin, and intermediate filaments, have been reported. An unusual feature is the existence of an extensive actin-myosin network forming a polygonal array.81,82,83
Unlike the cells of the central zone, the germinative zone cells, located peripherally on a whole-mount preparation, actively divide (see Fig. 15). In sagittal section (see Fig. 15), where they are located pre-equatorially, the cells are smaller and more cuboidal and contain more mitochondria than cells of the central zone.84 The cells are joined by a large number of lateral interdigitations (Fig. 16).
Following a terminal cell division, one or both of the daughter cells of the germinative zone are displaced into the adjacent transitional zone where differentiation is initiated. Mitosis is rarely found here, although it may occur in some pathologic conditions, such as intraocular inflammation.79
The cytoplasm of the cells in the transitional zone shows a large increase of ribosomes (polysomes) and an increase in the number of multivesicular bodies. Microtubules are also pronounced in these cells. Closer to the equator, the cells become more columnar and assume a pyramidal shape, the basal portion being wider than the apex.
LENS FIBERS
As the transitional zone cells continue to elongate, they queue up in columns known as the meridional rows, a precise register seen best on whole-mount preparations (see Fig. 15). In sagittal section, this corresponds to the region where the cells elongate and begin to internalize in the upper strata of the lens (see Fig. 15). They elongate by sending a basal process posteriorly beneath the capsule and the apex anteriorly beneath the epithelium. During the elongation process, the cell nucleus assumes a position more anterior than posterior in the cell. As the anterior termini of the cells extend toward the suture, the nuclei are positioned anterior to those of the more superficial cells. This progressive anterior shift of the cell nuclei, as the fibers internalize, collectively produces the pattern known as the lens bow (Fig. 17).
The cells composing the superficial bow region are filled with free ribosomes, polysomes, and rough endoplasmic reticulum. Cilia projecting from the apices are not uncommon, and basal bodies of cilia are frequently seen in the cytoplasm. As the cells of the bow region elongate, the nuclei assume the long slender shape of the fibers (Figs. 18 AND 19). During this process, the nucleoli increase in size85,86,87; at the light microscopic level, the cytoplasm stains intensely with the thiazine dyes (see Fig. 17), a basophilia attributed to an exaggerated ribosomal content.88,89 Both the nucleolar changes and the ribosomal increase reflect the characteristically elevated protein synthesis of the cells of this region.90 The subcapsular basal cytoplasm contains a large number of mitochondria and multivesicular bodies. Several types of cytoskeletal elements (see Fig. 18) appear in abundance in these cells: microtubules (about 25 nm in diameter),91 microfilaments (approximately 5 nm in diameter),92,93 and intermediate fibrils (approximately 10 nm in diameter).93,94 These cytoskeletal structures, which for the most part align parallel to the long axis of the cell, appear to play an important role in differentiation and morphogenesis of the lens fiber.95,96
Electron-microscopic analyses of the area of the meridional rows (i.e., where the lens fibers begin to internalize) reveal that adjacent cells enter the region in a staggered fashion, producing potential furrows between alternating cells (Fig. 20). This cadre may be responsible for the precise alignment of the cells as they enter the region. It has been hypothesized97 that the tension of the overlying capsule, in conjunction with the laterally placed and subjacent fibers, dictates the manner in which newly elongating cells align in the meridional rows. If this is indeed the case, it must then follow that an effect on any one of these parameters might result in “misdirected” fibergenesis and altered cytoarchitecture. It is interesting that the loss of meridional row alignment (Fig. 21) is associated with the development of a number of cataracts of varying causes in experimental animal,79,97,98 as well as in human,99 lenses. In the animal studies, it was shown that meridional row disorganization temporally precedes cataractogenesis. It remains to be determined whether there is a causal link between the loss of meridional row cytoarchitecture and the development of a number of types of cataract.
The differentiation of epithelial cells into fibers can occur at a remarkable rate, as indicated by animal studies.100,101,102,103,104 With the use of tritiated thymidine autoradiography, it has been shown that in the rat an epithelial cell becomes a mature lens fiber as deep as 0.4 mm into the lens substance within 5 weeks of labeling. Extrapolation suggests that during that period, approximately five new fiber layers were added each day. In older rats (1 year old) the rate is consistent with an average cellular deposition of one layer of cells per day; thus, the rate of fibergenesis, like that of mitosis, decreases with age.
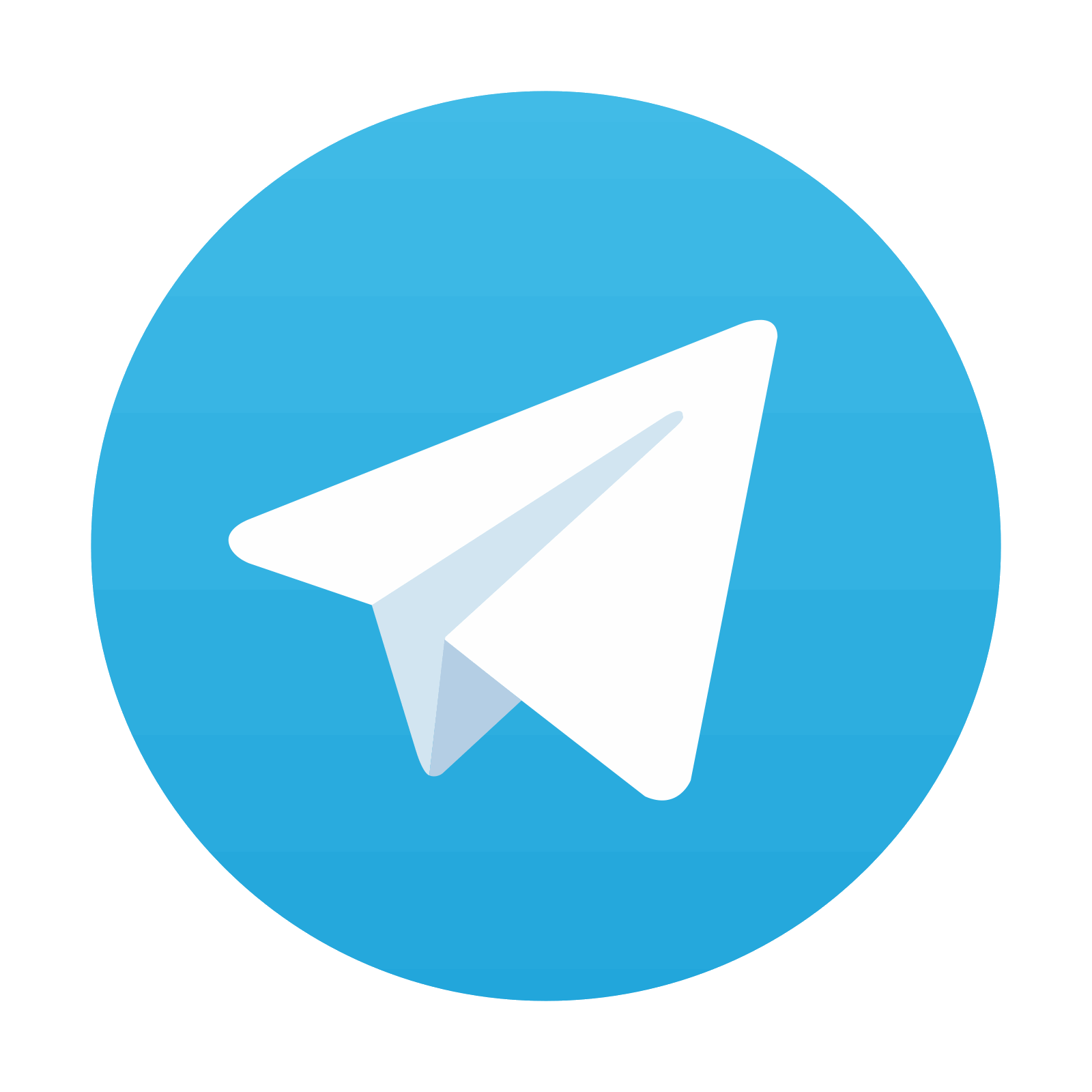
Stay updated, free articles. Join our Telegram channel
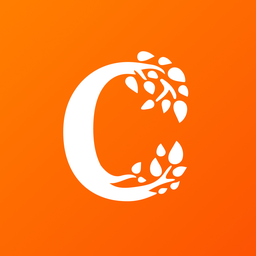
Full access? Get Clinical Tree
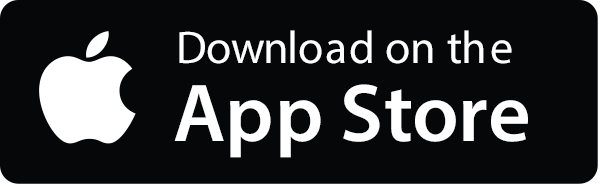
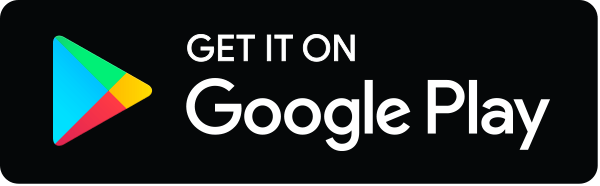