Introduction
Laser in situ keratomileusis (LASIK) is the most widely used technique to correct for most instances of spherocylindrical ametropia because of its safety and predictability, which lead to satisfactory outcomes for both patients and surgeons. Analysis of the US Food and Drug Administration (FDA) and multicenter data shows that modern lasers have significantly improved patient-reported visual outcomes after LASIK. It has been refined over the years and still undergoes continuous improvements. The LASIK surgical procedure consists of two important steps: the flap cut and the laser delivery.
LASIK aims at reshaping the corneal stroma after cutting and lifting a superficial flap in order to correct the preoperative refractive error. Each optical default has its own consequences in terms of geometrical reshaping, and these features have consequences on surgical parameters, such as flap dimension and thickness and the clinical outcomes. Important specific guidelines can help to optimize the visual outcomes of LASIK patients; these will be emphasized in this chapter.
Modern excimer laser programming software enables the surgeon to choose between various strategies of correction. Over time, the laser profiles of ablation, eye-tracking technologies, and delivery systems have been optimized to improve postoperative outcomes. LASIK surgery is based on the subtraction of a tissue lenticule, which is photoablated.
Differing from aspheric and customized wavefront-guided treatments, the profiles of ablation for conventional treatments do not take into account the asphericity of the cornea and the higher-order optical aberrations of the human eye. In most clinical situations, the main characteristics of the corneal reshaping are dictated by the correction of low-order (i.e., spectacle correctable) aberrations. Three-dimensional graphic representations of the theoretical characteristics of the corneal reshaping constraints, such as morphology of the lenticules ablated during LASIK for similar amounts of spherical and cylindrical treatment, can be generated using digital modeling software (see Appendix).
Pure Myopia ( )
The correction of myopic errors requires overall corneal flattening. The correction of pure myopia relies on the deliverance of a rotationally symmetric profile of ablation. The initial profile of ablation derived from the subtraction model of Munnerlyn et al. in 1988. In this model, the initial (preoperative) and final (postoperative) corneal surfaces are spherical and have different radii of curvature, the final surface being flatter ( Fig. 13.1 ). Conforming to that pioneering work, the change in paraxial corneal power can be predicted by considering the initial unablated and the final ablated corneal surface as two spherical surfaces, with a single but different radius of curvature ( Fig. 13.2 ). The removal of tissue is equivalent to adding a thin lens of equal but opposite power. No transition zone was proposed in this model, the junction between the optical zone and untouched periphery being of null thickness. The maximal depth of ablation is attained in the center of the optical zone (OZ), which is also the location of the minimal corneal pachymetry zone. From that mathematical model, a simple rule of thumb could be formulated: the maximal depth of ablation is roughly equal to the magnitude of treated myopia (in diopters) multiplied by the squared value of the OZ diameter (in millimeters) divided by 3 ( Fig. 13.2C ). This formula slightly underestimates the real depth of laser ablation, especially for higher magnitudes of treatment. While the precise maximal depth of ablation is given by the laser programming software, this simple formula enables the clinician to estimate the maximal depth of ablation from the clinical refraction.




LASIK for Low to Moderate Myopia
The LASIK technique for low to moderate myopia is safe and effective, and patient satisfaction is extremely high. With proper patient selection, the incidence of complication is low and rarely leads to severe visual loss. Night visual symptoms, reduced contrast sensitivity, and retreatment can lead to a decline in patient satisfaction with LASIK. Customized ablation using wavefront aberrometry and its optimized profiles were created to correct higher-order aberrations and give more vision quality to patients. Despite high visual demands, physicians and aviators having LASIK had a high percentage of good visual outcomes, satisfaction, and quality-of-life improvements. To date, there is no evident difference in both ocular low-order and higher-order aberrations and visual performance between photorefractive keratectomy (PRK) and LASIK. These results suggest that surgeons can choose refractive procedures according to corneal conditions or the daily activities of patients. In particular, patients with keratoconus or forme fruste keratoconus should not undergo LASIK. Night visual symptoms, reduced contrast sensitivity, and retreatment could have led to a decline in patient satisfaction with LASIK when it was performed with noncustomized profiles, which were designed on smaller optical zones in the late 1990s and early 2000s. Efforts to eliminate these variables have been achieved with wavefront aberrometry and custom ablations since then.
LASIK in High Myopia
Underlying keratectasia, less corneal thickness, and greater stromal ablation are the main risk factors of corneal ectasia after LASIK. Forme fruste keratoconus, genetic predisposition to keratoconus, low residual stromal bed thickness (through high myopia, thin preoperative cornea, or thick LASIK flap), and irregular corneal topography have been identified as risk factors for keratectasia development after refractive surgical procedures. Therefore any patient presenting clinical and/or topographic evidence of corneal abnormalities—such as keratoconus, forme fruste keratoconus, and pellucid marginal degeneration—should be excluded from LASIK surgery. In normal corneas, the depth of ablation is an important factor to consider, especially in patients having initially thin corneas and/or high levels of myopia. To reduce the risk of excessive corneal biomechanical weakening and to prevent iatrogenic keratectasia, there is consensus for the necessity of preserving a certain minimum thickness of untouched posterior stromal bed. The range of residual corneal thickness that puts the eye at risk for developing keratectasia is not precisely determined, but a minimum value between 250 and 300 µm is usually recommended. A residual stromal bed thickness of 250 µm does not preclude the development of keratectasia after LASIK. Other factors, such as slight asymmetric astigmatism or an isolated difference in central corneal thickness between eyes, may indicate an increased risk of corneal ectasia. Some authors have advocated the exclusion from LASIK of patients with thin cornea (< 490 µm) despite normal topography and low refractive error. A newly proposed metric, percentage tissue altered (PTA), has been reported to be an indicator for post-LASIK ectasia risk calculation.
To increase the safety of LASIK for high myopia by maintaining a posterior stromal bed of sufficient thickness, the following strategies should be considered.
Reducing the Magnitude of the Treatment (Intended Undercorrection)
Significant undercorrection is generally poorly accepted, especially by young (nonpresbyopic) patients, but can be part of a monovision strategy in presbyopic patients. Because the difference of refraction must be limited to approximately 1.50 D in monovision strategies, this will have limited effect on the depth of ablation.
Reducing the Programmed Optical Zone
Because the depth of the treatment is proportional to the square of the diameter of the OZ, reducing the latter can significantly reduce the depth of ablation. For example, reducing the OZ diameter from 6 mm to 5 mm for the same magnitude of treatment induces a reduction of nearly 30% of the maximal depth of ablation (and would also reduce dramatically the volume of the ablated lenticule). Thus reducing the OZ could theoretically result in an important decrease of ablation depth and allow the full correction of high myopic errors (8 D and above) in patients with normal or thin corneas. However, many studies have shown that excessive reduction of the ablation zone diameter can lead to poor optical quality and increased risk of regression of the refractive effect. Even if there is no abrupt step in the periphery of the treated zone, there is an important gradient of curvature due to the law of curvature conservation. This rapid steepening causes the peripheral rays to bend excessively (spherical aberration). Flattening the central corneal area causes an increase of curvature at the junction of the optical and transition zones. This curvature change can be spread further out in the periphery by realizing a transition zone ( Figs. 13.3A and 13.3B ). However, the realization of a large transition zone implies an increase in the central depth of ablation. This may be counterintuitive to the clinician because of the usual color scale representation of the corneal curvature with specular topography ( Fig. 13.4C ). The OZ (flatter area) corresponds postoperatively to a blue zone, whereas the area of progressive steepening corresponds to a reddish zone. Enlarging the OZ (pushing out the red ring) would not be achieved by additional spots on the OZ periphery alone, which would result in a steepening of the central cornea (hyperopic-like ablation). The algorithms that preside for the design of the transition zone are proprietary, and there is a lack of theoretical data in the literature to address the theoretical issues of particular transition zone designs. Some authors have suggested avoiding abrupt curvature changes at the OZ periphery of the optical zone by using special transition profiles at the price of a slight reduction of the OZ diameter. When astigmatism is associated with myopia (compound myopic astigmatism), the reduction of the OZ diameter can be achieved selectively on the steeper initial meridians, with no modification on the flat meridian. This would result in an elliptical OZ whose ablation maximal depth is reduced as compared to a circular one. Using the relation between depth and magnitude of myopic correction, the desired selective correction can be applied over the corneal meridians for a given central depth of ablation ( Fig. 13.4 ).




Use of Multizone/Multipass or Aspheric Profiles of Ablation
Theoretical modeling studies show that customizing the myopic profile of ablation to control for the postoperative profile asphericity has significant effects on the maximal depth of ablation. Aiming at increased postoperative prolateness by targeting a more negative asphericity (or reduced postoperative oblateness) incurs an increase in the maximal depth of ablation as compared to that of a spherical Munnerlyn-based treatment ( Fig. 13.5 ). Conversely, aiming at reduced postoperative prolateness (or increased postoperative prolateness) results in reduction of the maximal depth of ablation. Many clinical studies have shown that after conventional myopic LASIK or PRK, the postoperative corneal contour within the OZ conforms to an oblate ellipse. This oblate shift is proportional to the magnitude of treatment and is influenced by neither the initial asphericity nor the apical corneal curvature. We have first demonstrated that this oblate shift is opposite to the theoretical predictions of finite models that predict that the corneal profile should be more prolate within the OZ for initially prolate corneas. Explanatory reasons for this discrepancy are numerous (biological remodeling, biomechanical effect, reduced laser ablation due to peripheral corneal obliquity). Thus using an aspheric profile of ablation to reduce the postoperative prolateness (or increase postoperative oblateness) may have unpredictable effects and result in an excessive oblateness with marked reduction of the functional OZ and poor optical quality at large pupil diameters ( Fig. 13.6 ). Similar limitations have applied for the multizone–multipass profiles of ablation that were effective in reducing the depth of ablation but at the price of the reduction of the functional OZ.


Reducing the Thickness of the Corneal Flap
Despite dissimilarities between different platforms, all femtosecond (FS) lasers are predictable and safe for making corneal flaps in LASIK. By adjusting the depth of the infrared ultrashort pulse focus, the surgeon is offered a wider range of flap thickness, with a better predictability (reduced standard deviation).
The flap obtained with the FS laser is relatively independent of the operated cornea and patient characteristics ( Fig. 13.7 ). This is opposed to microkeratome LASIK surgery, in which there were factors such as preoperative total corneal pachymetry, age, preoperative keratometry, and microkeratome suction duration correlated to the flap thickness. With microkeratome flap cuts, significant standard deviation between the predicted and obtained flap thickness is confirmed by several authors and the flap thickness was not uniform over the flap area. Some reports of keratectasia following LASIK, despite safe residual bed thickness calculation, may have resulted from larger variation in flap thickness ( Fig. 13.8 ). These results emphasized the importance of measuring flap thickness and corneal bed thickness during surgery, using contact or noncontact methods. Flap-thickness values can be calculated using intraoperative optical pachymetry, but they were significantly lower than programmed values or OCT measurements performed in the postoperative period.





LASIK for Low to Moderate Myopia
The LASIK technique for low to moderate myopia is safe and effective, and patient satisfaction is extremely high. With proper patient selection, the incidence of complication is low and rarely leads to severe visual loss. Night visual symptoms, reduced contrast sensitivity, and retreatment can lead to a decline in patient satisfaction with LASIK. Customized ablation using wavefront aberrometry and its optimized profiles were created to correct higher-order aberrations and give more vision quality to patients. Despite high visual demands, physicians and aviators having LASIK had a high percentage of good visual outcomes, satisfaction, and quality-of-life improvements. To date, there is no evident difference in both ocular low-order and higher-order aberrations and visual performance between photorefractive keratectomy (PRK) and LASIK. These results suggest that surgeons can choose refractive procedures according to corneal conditions or the daily activities of patients. In particular, patients with keratoconus or forme fruste keratoconus should not undergo LASIK. Night visual symptoms, reduced contrast sensitivity, and retreatment could have led to a decline in patient satisfaction with LASIK when it was performed with noncustomized profiles, which were designed on smaller optical zones in the late 1990s and early 2000s. Efforts to eliminate these variables have been achieved with wavefront aberrometry and custom ablations since then.
LASIK in High Myopia
Underlying keratectasia, less corneal thickness, and greater stromal ablation are the main risk factors of corneal ectasia after LASIK. Forme fruste keratoconus, genetic predisposition to keratoconus, low residual stromal bed thickness (through high myopia, thin preoperative cornea, or thick LASIK flap), and irregular corneal topography have been identified as risk factors for keratectasia development after refractive surgical procedures. Therefore any patient presenting clinical and/or topographic evidence of corneal abnormalities—such as keratoconus, forme fruste keratoconus, and pellucid marginal degeneration—should be excluded from LASIK surgery. In normal corneas, the depth of ablation is an important factor to consider, especially in patients having initially thin corneas and/or high levels of myopia. To reduce the risk of excessive corneal biomechanical weakening and to prevent iatrogenic keratectasia, there is consensus for the necessity of preserving a certain minimum thickness of untouched posterior stromal bed. The range of residual corneal thickness that puts the eye at risk for developing keratectasia is not precisely determined, but a minimum value between 250 and 300 µm is usually recommended. A residual stromal bed thickness of 250 µm does not preclude the development of keratectasia after LASIK. Other factors, such as slight asymmetric astigmatism or an isolated difference in central corneal thickness between eyes, may indicate an increased risk of corneal ectasia. Some authors have advocated the exclusion from LASIK of patients with thin cornea (< 490 µm) despite normal topography and low refractive error. A newly proposed metric, percentage tissue altered (PTA), has been reported to be an indicator for post-LASIK ectasia risk calculation.
To increase the safety of LASIK for high myopia by maintaining a posterior stromal bed of sufficient thickness, the following strategies should be considered.
Reducing the Magnitude of the Treatment (Intended Undercorrection)
Significant undercorrection is generally poorly accepted, especially by young (nonpresbyopic) patients, but can be part of a monovision strategy in presbyopic patients. Because the difference of refraction must be limited to approximately 1.50 D in monovision strategies, this will have limited effect on the depth of ablation.
Reducing the Programmed Optical Zone
Because the depth of the treatment is proportional to the square of the diameter of the OZ, reducing the latter can significantly reduce the depth of ablation. For example, reducing the OZ diameter from 6 mm to 5 mm for the same magnitude of treatment induces a reduction of nearly 30% of the maximal depth of ablation (and would also reduce dramatically the volume of the ablated lenticule). Thus reducing the OZ could theoretically result in an important decrease of ablation depth and allow the full correction of high myopic errors (8 D and above) in patients with normal or thin corneas. However, many studies have shown that excessive reduction of the ablation zone diameter can lead to poor optical quality and increased risk of regression of the refractive effect. Even if there is no abrupt step in the periphery of the treated zone, there is an important gradient of curvature due to the law of curvature conservation. This rapid steepening causes the peripheral rays to bend excessively (spherical aberration). Flattening the central corneal area causes an increase of curvature at the junction of the optical and transition zones. This curvature change can be spread further out in the periphery by realizing a transition zone ( Figs. 13.3A and 13.3B ). However, the realization of a large transition zone implies an increase in the central depth of ablation. This may be counterintuitive to the clinician because of the usual color scale representation of the corneal curvature with specular topography ( Fig. 13.4C ). The OZ (flatter area) corresponds postoperatively to a blue zone, whereas the area of progressive steepening corresponds to a reddish zone. Enlarging the OZ (pushing out the red ring) would not be achieved by additional spots on the OZ periphery alone, which would result in a steepening of the central cornea (hyperopic-like ablation). The algorithms that preside for the design of the transition zone are proprietary, and there is a lack of theoretical data in the literature to address the theoretical issues of particular transition zone designs. Some authors have suggested avoiding abrupt curvature changes at the OZ periphery of the optical zone by using special transition profiles at the price of a slight reduction of the OZ diameter. When astigmatism is associated with myopia (compound myopic astigmatism), the reduction of the OZ diameter can be achieved selectively on the steeper initial meridians, with no modification on the flat meridian. This would result in an elliptical OZ whose ablation maximal depth is reduced as compared to a circular one. Using the relation between depth and magnitude of myopic correction, the desired selective correction can be applied over the corneal meridians for a given central depth of ablation ( Fig. 13.4 ).




Use of Multizone/Multipass or Aspheric Profiles of Ablation
Theoretical modeling studies show that customizing the myopic profile of ablation to control for the postoperative profile asphericity has significant effects on the maximal depth of ablation. Aiming at increased postoperative prolateness by targeting a more negative asphericity (or reduced postoperative oblateness) incurs an increase in the maximal depth of ablation as compared to that of a spherical Munnerlyn-based treatment ( Fig. 13.5 ). Conversely, aiming at reduced postoperative prolateness (or increased postoperative prolateness) results in reduction of the maximal depth of ablation. Many clinical studies have shown that after conventional myopic LASIK or PRK, the postoperative corneal contour within the OZ conforms to an oblate ellipse. This oblate shift is proportional to the magnitude of treatment and is influenced by neither the initial asphericity nor the apical corneal curvature. We have first demonstrated that this oblate shift is opposite to the theoretical predictions of finite models that predict that the corneal profile should be more prolate within the OZ for initially prolate corneas. Explanatory reasons for this discrepancy are numerous (biological remodeling, biomechanical effect, reduced laser ablation due to peripheral corneal obliquity). Thus using an aspheric profile of ablation to reduce the postoperative prolateness (or increase postoperative oblateness) may have unpredictable effects and result in an excessive oblateness with marked reduction of the functional OZ and poor optical quality at large pupil diameters ( Fig. 13.6 ). Similar limitations have applied for the multizone–multipass profiles of ablation that were effective in reducing the depth of ablation but at the price of the reduction of the functional OZ.


Reducing the Thickness of the Corneal Flap
Despite dissimilarities between different platforms, all femtosecond (FS) lasers are predictable and safe for making corneal flaps in LASIK. By adjusting the depth of the infrared ultrashort pulse focus, the surgeon is offered a wider range of flap thickness, with a better predictability (reduced standard deviation).
The flap obtained with the FS laser is relatively independent of the operated cornea and patient characteristics ( Fig. 13.7 ). This is opposed to microkeratome LASIK surgery, in which there were factors such as preoperative total corneal pachymetry, age, preoperative keratometry, and microkeratome suction duration correlated to the flap thickness. With microkeratome flap cuts, significant standard deviation between the predicted and obtained flap thickness is confirmed by several authors and the flap thickness was not uniform over the flap area. Some reports of keratectasia following LASIK, despite safe residual bed thickness calculation, may have resulted from larger variation in flap thickness ( Fig. 13.8 ). These results emphasized the importance of measuring flap thickness and corneal bed thickness during surgery, using contact or noncontact methods. Flap-thickness values can be calculated using intraoperative optical pachymetry, but they were significantly lower than programmed values or OCT measurements performed in the postoperative period.





Reducing the Magnitude of the Treatment (Intended Undercorrection)
Significant undercorrection is generally poorly accepted, especially by young (nonpresbyopic) patients, but can be part of a monovision strategy in presbyopic patients. Because the difference of refraction must be limited to approximately 1.50 D in monovision strategies, this will have limited effect on the depth of ablation.
Reducing the Programmed Optical Zone
Because the depth of the treatment is proportional to the square of the diameter of the OZ, reducing the latter can significantly reduce the depth of ablation. For example, reducing the OZ diameter from 6 mm to 5 mm for the same magnitude of treatment induces a reduction of nearly 30% of the maximal depth of ablation (and would also reduce dramatically the volume of the ablated lenticule). Thus reducing the OZ could theoretically result in an important decrease of ablation depth and allow the full correction of high myopic errors (8 D and above) in patients with normal or thin corneas. However, many studies have shown that excessive reduction of the ablation zone diameter can lead to poor optical quality and increased risk of regression of the refractive effect. Even if there is no abrupt step in the periphery of the treated zone, there is an important gradient of curvature due to the law of curvature conservation. This rapid steepening causes the peripheral rays to bend excessively (spherical aberration). Flattening the central corneal area causes an increase of curvature at the junction of the optical and transition zones. This curvature change can be spread further out in the periphery by realizing a transition zone ( Figs. 13.3A and 13.3B ). However, the realization of a large transition zone implies an increase in the central depth of ablation. This may be counterintuitive to the clinician because of the usual color scale representation of the corneal curvature with specular topography ( Fig. 13.4C ). The OZ (flatter area) corresponds postoperatively to a blue zone, whereas the area of progressive steepening corresponds to a reddish zone. Enlarging the OZ (pushing out the red ring) would not be achieved by additional spots on the OZ periphery alone, which would result in a steepening of the central cornea (hyperopic-like ablation). The algorithms that preside for the design of the transition zone are proprietary, and there is a lack of theoretical data in the literature to address the theoretical issues of particular transition zone designs. Some authors have suggested avoiding abrupt curvature changes at the OZ periphery of the optical zone by using special transition profiles at the price of a slight reduction of the OZ diameter. When astigmatism is associated with myopia (compound myopic astigmatism), the reduction of the OZ diameter can be achieved selectively on the steeper initial meridians, with no modification on the flat meridian. This would result in an elliptical OZ whose ablation maximal depth is reduced as compared to a circular one. Using the relation between depth and magnitude of myopic correction, the desired selective correction can be applied over the corneal meridians for a given central depth of ablation ( Fig. 13.4 ).




Use of Multizone/Multipass or Aspheric Profiles of Ablation
Theoretical modeling studies show that customizing the myopic profile of ablation to control for the postoperative profile asphericity has significant effects on the maximal depth of ablation. Aiming at increased postoperative prolateness by targeting a more negative asphericity (or reduced postoperative oblateness) incurs an increase in the maximal depth of ablation as compared to that of a spherical Munnerlyn-based treatment ( Fig. 13.5 ). Conversely, aiming at reduced postoperative prolateness (or increased postoperative prolateness) results in reduction of the maximal depth of ablation. Many clinical studies have shown that after conventional myopic LASIK or PRK, the postoperative corneal contour within the OZ conforms to an oblate ellipse. This oblate shift is proportional to the magnitude of treatment and is influenced by neither the initial asphericity nor the apical corneal curvature. We have first demonstrated that this oblate shift is opposite to the theoretical predictions of finite models that predict that the corneal profile should be more prolate within the OZ for initially prolate corneas. Explanatory reasons for this discrepancy are numerous (biological remodeling, biomechanical effect, reduced laser ablation due to peripheral corneal obliquity). Thus using an aspheric profile of ablation to reduce the postoperative prolateness (or increase postoperative oblateness) may have unpredictable effects and result in an excessive oblateness with marked reduction of the functional OZ and poor optical quality at large pupil diameters ( Fig. 13.6 ). Similar limitations have applied for the multizone–multipass profiles of ablation that were effective in reducing the depth of ablation but at the price of the reduction of the functional OZ.


Reducing the Thickness of the Corneal Flap
Despite dissimilarities between different platforms, all femtosecond (FS) lasers are predictable and safe for making corneal flaps in LASIK. By adjusting the depth of the infrared ultrashort pulse focus, the surgeon is offered a wider range of flap thickness, with a better predictability (reduced standard deviation).
The flap obtained with the FS laser is relatively independent of the operated cornea and patient characteristics ( Fig. 13.7 ). This is opposed to microkeratome LASIK surgery, in which there were factors such as preoperative total corneal pachymetry, age, preoperative keratometry, and microkeratome suction duration correlated to the flap thickness. With microkeratome flap cuts, significant standard deviation between the predicted and obtained flap thickness is confirmed by several authors and the flap thickness was not uniform over the flap area. Some reports of keratectasia following LASIK, despite safe residual bed thickness calculation, may have resulted from larger variation in flap thickness ( Fig. 13.8 ). These results emphasized the importance of measuring flap thickness and corneal bed thickness during surgery, using contact or noncontact methods. Flap-thickness values can be calculated using intraoperative optical pachymetry, but they were significantly lower than programmed values or OCT measurements performed in the postoperative period.





Pure Hyperopia ( )
In comparison to myopic correction, in which the goal is to flatten the central cornea, in hyperopia the central corneal area is to be steepened to increase its optical power ( Fig. 13.9 ). This central steepening makes the planned correction of the hyperopic eye more difficult, because the steepened central corneal portion must join the peripheral unablated area of lower curvature via a transition area. These represent the important special features of the correction of hyperopic errors that we will emphasize in this chapter.

The profile of ablation to correct for spherical hyperopia is radially symmetric and predominates in the periphery in an annular fashion. A subtraction shape model based on geometric optics allowed Munnerlyn et al. to announce in 1988 the principles of laser-guided photoablation in the central corneal area (effective OZ). Spherical hyperopic ablation results in the ablation of a concave lenticule within the OZ ( Fig. 13.10 ). Its thickness, null in the center, increases progressively toward the periphery where it reaches its maximum at the edge of the OZ. In first-order approximation, the maximum thickness of the edge of the ablated lenticule over the OZ is proportional to the magnitude of the hyperopic treatment and to the square of the chosen OZ diameter. The volume of tissue ablation needed to steepen the cornea is thus limited by the initial anterior surface and the final postoperative steeper spherical surface over a circular optical zone.

For the necessary geometric feature, any cornea that had tissue removed centrally to steepen its curvature (OZ) while leaving the periphery untouched must undergo an additional ablation to sculpt a smooth blending zone. This flatter area, commonly referred to as the transition zone, thus represents a constant feature that ideally would have no undesirable optical effects and would ensure the stability of the induced refractive changes in the OZ by limiting unwanted biologic and biomechanical changes.
When we address theoretical considerations of the different approaches to blending a steepened OZ to the untouched peripheral cornea, some constraints must be taken into consideration ( Fig. 13.11 ). Because the patterns of ablation for the hyperopic optical and transition zones are proprietary, there are insufficient data to compare one ablation profile with another. Ideally, these profiles should have characteristics that limit the compensatory epithelial hyperplasia in annular midperipheral ablation. In LASIK, the corneal flap covering of the ablation zone minimizes the epithelial healing response. This might account for the better results reported for this technique over PRK. The total ablation zone diameter being equal to the outer diameter of the transition zone, obtaining large flap sizes is mandatory for hyperopic LASIK procedures ( Fig. 13.12 ). Modern FS laser platforms enable the surgeon to program and obtain flaps whose diameter can reach theoretically up to 10 mm ( Fig. 13.13 ). Some publications have emphasized the need for a large transition zone outer diameter in order to improve the biologic tolerance and minimize regression. Conversely, enlarging the OZ diameter, although desirable to preserve the quality of vision and reduce the risk of decentration, represents a limiting factor because the depth per diopter at the edge of the OZ will increase with the square of the OZ diameter. This could account for the low success rate observed for corrections over 5 D or 6 D of hyperopia. A large cutting gives an increased risk of some intraoperative complications, such as intraoperative bleeding, intraoperative epithelial defect, and blood in the interface.



Outcomes of Hyperopic LASIK
The factor that negatively influences the outcome of hyperopic LASIK is the degree of hyperopia corrected. Better outcomes are expected when the magnitude of hyperopia is equal to or less than +4 D. Preoperative keratometry does not seem to significantly influence the postoperative results, and postoperative keratometry (> 48 D) does not result in significant worsening of visual results when the attempted correction is less than +4 D.
Dry eye is problematic after LASIK for hyperopia and is associated with refractive regression ( Fig. 13.14 ). Chronic dry eye is associated with preoperative dry-eye symptoms; female gender ; and a narrow, superiorly placed hinge flap. Preoperative and postoperative treatments—such as a combination of artificial tears, topical autologous serum, topical cyclosporine A, and punctual occlusion—can help in effectively managing patients with severe dry eye.

The quality of vision after hyperopic LASIK and PRK has been investigated. Clinical studies show that third and higher total and corneal aberrations increase significantly after hyperopic LASIK. The largest increase occurs in spherical aberration, which shifts to negative value. This is due to postoperative exaggeration of the negative corneal asphericity (increased postoperative prolateness) after hyperopic profile delivery ( Fig. 13.15 ). As for myopia, there are some discrepancies between these findings and the theoretical predictions using aspheric corneal models and Munnerlyn-based treatments. Reasons to explain those discrepancies are similar to those invoked for myopic profiles. Wound healing might be an especially important issue due to the characteristics of corneal reshaping after hyperopic LASIK. Optimal centration on the entrance pupil is also critical to avoid the induction of odd higher-order aberrations, such as trefoil, third-order, and fifth-order coma ( Fig. 13.16 ). Many reports suggest that the optimal centration should be directed onto or toward the corneal vertex (first Purkinje reflex or coaxial light reflex; Fig. 13.17 ). Efficient eye tracking, aspheric ablation profiles, and wavefront-supported customized ablation may improve the results of hyperopic LASIK.





Presbyopia usually has earlier onset in the hyperopic population than in the myopic population. Many hyperopic patients are referred for refractive surgery counseling in their forties or fifties because of the global visual impairment owing to near and distance poor uncorrected visual acuity. Monovision seems to lead to results in hyperopes that are slightly inferior to results in the myopic population. The increased corneal multifocality can be used to increase the depth of focus to presbyopic patients.
Outcomes of Hyperopic LASIK
The factor that negatively influences the outcome of hyperopic LASIK is the degree of hyperopia corrected. Better outcomes are expected when the magnitude of hyperopia is equal to or less than +4 D. Preoperative keratometry does not seem to significantly influence the postoperative results, and postoperative keratometry (> 48 D) does not result in significant worsening of visual results when the attempted correction is less than +4 D.
Dry eye is problematic after LASIK for hyperopia and is associated with refractive regression ( Fig. 13.14 ). Chronic dry eye is associated with preoperative dry-eye symptoms; female gender ; and a narrow, superiorly placed hinge flap. Preoperative and postoperative treatments—such as a combination of artificial tears, topical autologous serum, topical cyclosporine A, and punctual occlusion—can help in effectively managing patients with severe dry eye.

The quality of vision after hyperopic LASIK and PRK has been investigated. Clinical studies show that third and higher total and corneal aberrations increase significantly after hyperopic LASIK. The largest increase occurs in spherical aberration, which shifts to negative value. This is due to postoperative exaggeration of the negative corneal asphericity (increased postoperative prolateness) after hyperopic profile delivery ( Fig. 13.15 ). As for myopia, there are some discrepancies between these findings and the theoretical predictions using aspheric corneal models and Munnerlyn-based treatments. Reasons to explain those discrepancies are similar to those invoked for myopic profiles. Wound healing might be an especially important issue due to the characteristics of corneal reshaping after hyperopic LASIK. Optimal centration on the entrance pupil is also critical to avoid the induction of odd higher-order aberrations, such as trefoil, third-order, and fifth-order coma ( Fig. 13.16 ). Many reports suggest that the optimal centration should be directed onto or toward the corneal vertex (first Purkinje reflex or coaxial light reflex; Fig. 13.17 ). Efficient eye tracking, aspheric ablation profiles, and wavefront-supported customized ablation may improve the results of hyperopic LASIK.





Presbyopia usually has earlier onset in the hyperopic population than in the myopic population. Many hyperopic patients are referred for refractive surgery counseling in their forties or fifties because of the global visual impairment owing to near and distance poor uncorrected visual acuity. Monovision seems to lead to results in hyperopes that are slightly inferior to results in the myopic population. The increased corneal multifocality can be used to increase the depth of focus to presbyopic patients.
Simple, Compound, and Mixed Astigmatic Errors ( )
Myopia is often associated with some degree of astigmatism in the general population. It is estimated that astigmatism of more than 0.50 D is present in 44.4% of the population and that 8.44% of these subjects have astigmatism of 1.50 D or more.
Regular astigmatism is mainly generated by excessive corneal toricity. Corneal toricity can be suppressed either by flattening the steepest meridians to the curvature of the initially flatter meridian or by steepening the flattest meridians to the curvature of the initially steeper meridian. LASIK for astigmatism aims at reducing this excessive toricity by etching from the corneal surface an adequate toric lenticule of corneal tissue of variable thickness. Pure positive and negative cylindrical excimer laser treatments are based on the combination of three elementary profiles of ablation selectively delivered on the different corneal meridians ( Fig. 13.18 ).



Simple myopic and hyperopic astigmatic treatments rely on the use of negative and positive cylinder modes, respectively. Compound and mixed astigmatism are treated by the combination of negative and/or positive cylindrical and spherical modes.
Simple Astigmatism
Simple Hyperopic Astigmatism
This is optimally treated by using positive cylinder excimer ablation. This mode consists of steepening the flattest meridian to the desired value. Three-dimensional modeling is useful to conceptualize its characteristics and that of the required blend zone along the flat axis ( Fig. 13.19 ). No significant coupling effect on the spherical component has been observed after pure positive cylindrical treatments; this relates essentially to the geometry of this profile that spares the center of the cornea and the steepest initial meridian. A blend zone is necessary to blend the abrupt ablation created at the periphery of the OZ along the initial flattest meridians. Ideally, this blend zone takes an elliptical perimeter, the longest axis of which has the direction of the initially flat axis. When dealing with compound hyperopic astigmatism or mixed astigmatism, using the expression of refraction that incurs the maximum magnitude, positive cylinder mode results in the minimum amount of tissue ablation. Negative cylinder ablation was proposed earlier than positive cylinder mode because of the engineering constraints of the first delivery systems, which used slits and diaphragms. The use of negative cylindrical modes to treat for pure or compound hyperopic astigmatism led to initial mitigated reported results because of increased tissue ablation leading to excessive scarring and undesired refractive shifts.


Simple Myopic Astigmatism
Pure cylindrical myopic ablation consists of ablating a lenticule with a convex shape along the initial steeper meridian and with constant thickness along the initial flatter meridian in order to preserve its curvature ( Fig. 13.20 ). Because of this latter constraint, the amount of pure cylinder treatment is superior to the amount of spherical treatment for a given degree of negative dioptric treatment. The maximal thickness of the myopic cylindrical ablated lenticule is located along the flatter meridian and is identical to that of a spherical myopic lenticule for a given magnitude of treatment. This ablation pattern along the flat meridian is equivalent to that of a plano ablation ( Fig. 13.21 ). This plano ablation induces some flattening in the flattest meridian. This explains the frequent unanticipated effects from the optical and engineering perspectives of negative cylindrical treatment, such as undercorrection and hyperopic shift. To minimize the intensity of these phenomena, the edges of the ablation over the OZ have to be smoothed out evenly by enlarging the transition along the initially flatter meridian, thus creating an elliptical perimeter for the total ablation zone.



Relation Between Negative and Positive Cylindrical Ablations
Because the initially steeper principal meridian cannot be flattened selectively (this would imply plano ablation on the other principal meridian), the lenticule ablated for cylindrical myopic treatment can be considered as a combination of two successive treatments : cylindrical positive treatment of equal magnitude on the initially flatter meridian (to steepen its curvature until it equals that of the opposite principal meridian), followed by myopic spherical treatment of similar magnitude aiming to flatten both meridians ( Fig. 13.22 ). The final surface is spherical and its radius is equal to that of the initially flatter meridian. For example, a cylindrical treatment −1.00 × 180 degrees is equivalent in terms of the optical results and the amount of ablated tissue to the following sequential treatment: +1.00 × 90 degrees and −1. This additive relation is useful to compare strategies employed to treat compound and mixed astigmatism. The photoablated volume according to a particular sequential strategy can be converted in a particular sum of negative or positive spherical lenticules and positive cylindrical lenticules. In the past, before the approval of positive cylinder correction by the FDA in 2000, suboptimal strategies in terms of excessive photoablated volume incur negative cylinders and positive sphere sequential treatments. The negative sphere obtained from the splitting of the negative cylinder would then combine with the part of the positive spherical treatment to result in an unnecessary plano lenticule.


To compensate for the high reported rate of undercorrection of the cylindrical component and overcorrection of the spherical component with simple myopic astigmatic treatments, some strategies have been proposed by laser companies. In the case of sequential ablation, the strategy consists of anticipating both the undercorrection and the hyperopic shift by splitting the cylindrical treatment into two successive cylindrical ablations of opposite signs. Three-dimensional modeling is useful for understanding this concept ( Fig. 13.23 ). With most of the flying spot lasers, such compensation is integrated in the laser system and thus is not directly visible to the surgeon.


Compound Myopic, Hyperopic, and Mixed Astigmatism
Noncustom PRK or LASIK ablation of pure, compound, or mixed astigmatic refractive errors is based on paraxial models first described by Munnerlyn et al. They generally employ one or more of four elementary treatments: spherical myopic, spherical hyperopic, cylindrical myopic, and cylindrical hyperopic. Thus to achieve emmetropia, tissue photoablation within the OZ must yield a single final apical corneal curvature. As refraction (as commonly measured in clinical practice) is an arc-based mathematical expression limited to the principal major and minor axes, a compound astigmatic refractive error can be expressed by different equivalent expressions. Sequential treatment strategies for the correction of compound astigmatism were proposed, consisting of a combination of spherical and cylindrical treatments, as follows:
- •
ablating the cylinder along the flattest meridian and then treating the residual spherical component (positive cylinder approach);
- •
ablating the cylinder along the steepest meridian and then treating the residual spherical component (negative cylinder approach);
- •
ablating the total refractive error by two pure cylindrical ablations of opposite signs along the principal meridians without spherical correction (bitoric approach);
- •
ablating half the power of the cylinder along the steepest meridian and the remaining half along the flattest meridian, before treating the residual spherical equivalent (cross-cylinder approach).
Azar and Primack found that strategies combining hyperopic spherical and myopic cylindrical corrections incur the greatest amount of corneal tissue ablation. To provide a direct estimate of the difference in the amount of tissue ablation, we designed a method to compare and illustrate the amount of tissue ablation incurred by different sequential strategies using Boolean operations. This method confirmed the universal utility of combining plus cylindrical and negative or positive spherical ablations when treating any compound or mixed astigmatism while theoretically minimizing the volume of ablated corneal tissue. Regardless of the expressions chosen to program the correction (i.e., positive or negative cylinder format), the profile of ablation delivered with modern laser platforms equipped with flying-spot delivery systems is always the one that minimizes the amount of tissue removed. Using this approach, the magnitude of astigmatism to correct is expressed in the positive cylinder format of the refraction. It implies variable steepening of the corneal surface, aimed at reducing or suppressing the toricity of the anterior corneal surface, followed by a pure positive or negative spherical treatment to correct for the remaining defocus.
Hyperopic and Mixed Astigmatism
Negative cylindrical approaches to compound hyperopic or mixed astigmatism result in additional tissue ablation, the amount of which results from the combination of the myopic spherical treatment in the flat meridian (which is part of the negative cylindrical treatment) with a positive spherical lenticule (in both meridians; Fig. 13.24 ). They should not be used. This combination also occurs with the cross-cylindrical ablation strategy when it is used to correct mixed or compound hyperopic astigmatism. This strategy has been proposed to favor postoperative prolate asphericity and to reduce the overcorrection on nonprincipal meridians. In hyperopic astigmatism correction with broad or slit scanning laser systems, the cross-cylinder technique may also reduce the increase in corneal eccentricity, leading to a more physiologic corneal shape.





For mixed astigmatism, the positive cylindrical approach and bitoric ablation would theoretically incur the same minimum volume of tissue ablation ( Fig. 13.25 ). However, because of the global characteristics of the cylindrical profiles of ablation achieved with the newest delivery systems (wide elliptical transition zones), a bitoric strategy might be more practical to treat mixed astigmatism and reduce the rate of retreatment. The bitoric strategy has also been shown to reduce the hyperopic shift caused by the use of the negative cylindrical treatment by ablating more selectively along the extremities of the flat meridian (positive cylindrical treatment), thus preventing the flattening of the latter and avoiding a shift of refraction toward hyperopia. A significant percentage of eyes (67.8%) gained 1 or more of best corrected visual acuity (BCVA) after bitoric LASIK for mixed astigmatism in a study conducted by Albarran-Diego et al. This might be explained by reduction of image distortion.



Deliverance of the correction of mixed and hyperopic astigmatism with flying-spot laser obeys several constraints, among which is the necessity of minimizing the spatial density of the ablation pulse. This results in a nonsequential photoablation in which the correction of the astigmatism is not performed as the successive deliverance of positive cylinder and a negative sphere or cylindrical corrections but rather as an integrated “full correction.” However, bitoric mixed astigmatism ablative treatments may display nontrivial coupling effects. A recent study has shown interest in considering historical coupling adjustments when planning mixed astigmatism treatments to improve surgical outcomes.
Compound Myopic Astigmatism
Sequential Strategy
Conventional strategies used to correct compound myopic astigmatism are sequential: the spherical and cylindrical components of the refractive errors are treated successively, over a circular OZ. For compound myopic astigmatism (e.g., −3.00 [−2.00 × 90 degrees]), all available sequential strategies would theoretically lead to the same amount of tissue ablation because any negative cylindrical treatment (−2.00 × 90 degrees) can be split into a sequence combining a negative sphere (−2.00) and a pure cylindrical treatment (+2.00 × 0 degrees). Recombining the negative spherical components and the pure cylindrical treatment leads to the equivalent following refraction expressed in the positive cylinder format, −5.00 (+2.00 × 0 degrees).
Elliptical Strategy
The sequential approach is not the only treatment for compound myopic astigmatism. By using a patented elliptical method, VISX software allows the full myopic and astigmatic correction to be sculpted into the cornea in one smooth ablation. This is made possible by the narrowing of the OZ along the initially steeper meridians ( Fig. 13.26 ). The treatment of compound myopic astigmatism aims both to suppress the toricity and to flatten the corneal anterior surface over the effective optical zone: in the elliptical modality, astigmatic and myopic correction are achieved by varying the diameter in elliptical fashion, the narrowest diameter achieving the greatest flattening effect. The relative size of the major and minor axes of the elliptical cut depends on the ratio between the cylindrical and spherical magnitudes. Comparative clinical studies have shown that the elliptical method leads to a significant improvement in results in patients treated for myopic compound astigmatism, at least for the correction of the cylinder. The elliptical method has several theoretical advantages, such as a reduction in the maximal depth of ablation and the induction of a natural transition zone with no steep edges. It implies, however, a reduction of the OZ diameter along the initially steeper meridian, which could theoretically cause optical aberrations with pupil dilation in low-light conditions. Recently, a simplified formula was proposed to accurately approximate the volume of corneal tissue ablated within the optical zone during pure spherical corrections. However, no quantitative computations of the tissue removed during cylindrical corrections have been published.

Strategy to Optimize the Clinical Outcomes of Astigmatic Treatments
Because the spherocylindrical expression of astigmatic treatments is relatively more complex than that of pure spherical treatment, the refractive formula entered in laser software should be carefully checked before treatment. Most laser software displays a two-dimensional or three-dimensional representation of the elementary characteristics of the computed profile of ablation, whose elliptical shape must match the orientation expected from the cylinder axis. The longest axis of the ellipse is oriented along the flattest corneal meridian in all cases. The spatial orientation of the toric profiles of ablation requires proper alignment of the corneal surface relative to the delivery system in order to avoid undercorrections ( Fig. 13.27 ). Human eyes often undergo torsional movements about their axes depending on body position. Accurate laser delivery can be facilitated by the apposition of horizontal ink marks when the patient is in seated or supine position. The surgeon can then detect and compensate for some cyclotorsion during treatment by appropriate head rotations and repositioning. Newer ablation systems are equipped with sophisticated tracking systems that can improve the clinical outcomes by reducing the amount of decentration and rotation. Some early laser devices could detect eyeball rotations by recognizing and tracking limbal ink marks. Currently, nearly all modern excimer laser platforms are linked to an acquisition system that enables the recognition of fine iris details as seen by the tracking laser camera and the compensation of ocular cyclotorsion.

Owing to the desirable oval shape of the ablation in the presence of astigmatism to correct for, the hinge of the flap should be located perpendicular to the shorter diameter of the ablation zone, that is, the steeper meridian, to optimize the spatial concordance between the laser delivery area and the exposed stromal bed. Some FS laser platforms, such as the FS200 (Alcon Wavelight), enable the rotation of the hinge over 360 degrees and the design elliptical flaps ( Fig. 13.28 ).

Simple Astigmatism
Simple Hyperopic Astigmatism
This is optimally treated by using positive cylinder excimer ablation. This mode consists of steepening the flattest meridian to the desired value. Three-dimensional modeling is useful to conceptualize its characteristics and that of the required blend zone along the flat axis ( Fig. 13.19 ). No significant coupling effect on the spherical component has been observed after pure positive cylindrical treatments; this relates essentially to the geometry of this profile that spares the center of the cornea and the steepest initial meridian. A blend zone is necessary to blend the abrupt ablation created at the periphery of the OZ along the initial flattest meridians. Ideally, this blend zone takes an elliptical perimeter, the longest axis of which has the direction of the initially flat axis. When dealing with compound hyperopic astigmatism or mixed astigmatism, using the expression of refraction that incurs the maximum magnitude, positive cylinder mode results in the minimum amount of tissue ablation. Negative cylinder ablation was proposed earlier than positive cylinder mode because of the engineering constraints of the first delivery systems, which used slits and diaphragms. The use of negative cylindrical modes to treat for pure or compound hyperopic astigmatism led to initial mitigated reported results because of increased tissue ablation leading to excessive scarring and undesired refractive shifts.


Simple Myopic Astigmatism
Pure cylindrical myopic ablation consists of ablating a lenticule with a convex shape along the initial steeper meridian and with constant thickness along the initial flatter meridian in order to preserve its curvature ( Fig. 13.20 ). Because of this latter constraint, the amount of pure cylinder treatment is superior to the amount of spherical treatment for a given degree of negative dioptric treatment. The maximal thickness of the myopic cylindrical ablated lenticule is located along the flatter meridian and is identical to that of a spherical myopic lenticule for a given magnitude of treatment. This ablation pattern along the flat meridian is equivalent to that of a plano ablation ( Fig. 13.21 ). This plano ablation induces some flattening in the flattest meridian. This explains the frequent unanticipated effects from the optical and engineering perspectives of negative cylindrical treatment, such as undercorrection and hyperopic shift. To minimize the intensity of these phenomena, the edges of the ablation over the OZ have to be smoothed out evenly by enlarging the transition along the initially flatter meridian, thus creating an elliptical perimeter for the total ablation zone.


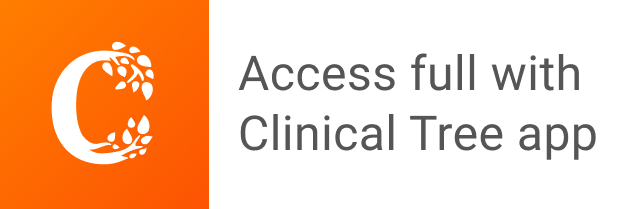