Fig. 1.1
Schematic of an OCT system based on low coherence interferometry using a Michelson interferometer design. Light from a broadband light source is divided by a beam splitter into the reference and sample arms. The reflected light from these two arms are recombined again through the beam splitter and detected with a photodetector. OCT images are produced by scanning the light in the sample arm to acquire sequential optical backscattering profiles along the axial direction. There are two types of OCT techniques, time-domain OCT (TD-OCT) and Fourier-domain OCT (FD-OCT), to reconstruct the intensity and time of flight of the backscattered light. In contrast to TD-OCT which uses a moving mirror in the reference arm, the reference mirror is stationary in FD-OCT, which includes spectral domain OCT and swept source OCT
Since the speed of light is much higher than that of sound waves, it is very challenging, if not impossible, to directly measure the time of flight for backscattered light. Instead, OCT is based on low coherence interferometry to measure the time of flight indirectly from optical interference generated by mixing the backscattered light with light from a reference mirror. A Michelson interferometer is most commonly employed in OCT systems. Light from the broadband light source is divided by a beam splitter into the reference and sample arms. The reflected light from these two arms are recombined again through the beam splitter and detected with a photodetector. Depending on the method used to reconstruct the intensity and time of flight of backscattered light, there are two types of OCT techniques, namely TD-OCT and FD-OCT.
In a TD-OCT system, a moving mirror is placed in the reference arm, and a single photodetector is employed. As the reference mirror in an OCT system moves along the axial direction, a corresponding depth-dependent backscattering profile of the sample is acquired. The axial resolution of OCT is determined by the coherence length of the light source, while the imaging speed is determined by how fast the reference mirror moves. Due to the relatively slow speed of the mechanical reference mirror, the imaging speed of commercial ophthalmic TD-OCT has been limited to 400 A-scans per second [9].
To further increase the imaging speed and the sensitivity, SD-OCT based on a fast linear detector array was demonstrated for retinal imaging in vivo in the early 2000s [10]. In a SD-OCT system, the reference mirror is stationary instead of moving. The imaging speed is determined by the speed of the linear detector array, which can be higher than tens of thousands of A-scans per second [11], yielding a roughly 100× improvement over that achieved with TD-OCT systems. SD-OCT detects the interferogram as a function of wavelength (λ), in contrast to the direct detection of backscattering profile as a function of depth position of the reference mirror in TD-OCT. As illustrated in Fig. 1.2, the depth information, more precisely the depth difference between the reference mirror and the sample, is encoded as the frequency of the interferogram fringes. When the depth of the sample is near to that of the reference mirror, the frequency of interferogram fringes is low (Fig. 1.2a). And when the depth of the sample is far from that of the reference mirror, the frequency of interferogram fringes is high (Fig. 1.2b). Light backscattered from different depths along the axial direction are detected simultaneously using a linear detector array (Fig. 1.2c). By performing a Fourier transform on the detected interferogram in wavenumber (k), an A-scan profile can be readily acquired.


Fig. 1.2
Illustration of FD-OCT signal reconstruction. A-scans are generated by Fourier transforming the interferograms. (a) A low-frequency interferogram corresponds to a signal near the reference mirror. (b) A high-frequency interferogram corresponds to a signal far from the reference mirror. (c) OCT interferograms can have multiple frequency components comprised of signal from different depths as reconstructed in the A-scan profile
The higher speed and sensitivity of SD-OCT [2–4] has enabled volumetric imaging with better image quality. On the other hand, SD-OCT is subject to a signal-to-noise ratio (SNR) roll-off characteristic that occurs in the FD-OCT technique. As shown in Fig. 1.3, it is commonly observed in SD-OCT that the reconstructed sample signal intensity gets weaker when it is farther from the zero-delay position.


Fig. 1.3
Illustration of signal roll-off in SD-OCT. The spectral resolution of the interferograms is limited by the spectrometer linear detector array. Higher frequency interferograms suffer more signal attenuation. The amplitude of reconstructed A-scans is therefore depth-dependent in SD-OCT
This SNR roll-off is primarily caused by the limited capability of SD-OCT to resolve the interferogram fringes. In an SD-OCT system using a broadband light source such as a superluminescent diode (SLD), the interferogram is generated by the spectrometer in the detection arm, which spreads the light at different wavelengths to be recorded by a linear detector array. However, due to the finite number of pixels on the linear detector array, the light of different wavelengths that are very close to each other fall on the same pixel and gets digitized without distinction, as illustrated in Fig. 1.4.


Fig. 1.4
Illustration of the limitation in resolvability of different wavelength light on finite pixels in a spectral domain OCT spectrometer
As a consequence, the resolvability of interferogram fringes is degraded. Mathematically, this constitutes an integration effect of the interferogram over the continuous spectrum of the light source across each element of the linear detector array. In other words, the resolvability of the interferogram fringes is affected differently during detection depending on frequency. As shown in Fig. 1.3, the higher the fringe frequency is, the more it is attenuated and the weaker its amplitude becomes. Accordingly, after Fourier transform, the amplitude of the reconstructed signal will be depth dependent. It was reported that the SNR roll-off for SD-OCT can be as high as 15 dB/mm [12]. Considering the typical 2 mm imaging range for commercial ophthalmic OCT instrument, an SNR drop of up to 30 dB is very significant and it is not unusual that the lower end of the imaging range is practically unusable.
By eliminating the use of the spectrometer and the linear detector array, SS-OCT employs a rapid-wavelength tuning laser and high-speed photodetector and signal digitizer. SS-OCT thereby offers a significant solution to the limitation of spectrometer spectral resolution as seen in SD-OCT, and thus enables deeper range imaging without compromising the sensitivity at a deeper position caused by the above-mentioned SNR roll-off. As illustrated in Fig. 1.5, the instantaneous line width of the laser light source used in SS-OCT, rather than the finite pixel size of the linear detector array used in SD-OCT, primarily determines the resolvability of the interferogram fringes.


Fig. 1.5
Illustration of signal roll-off in SS-OCT. The tuning laser instantaneous line width and high-speed digitizer yields good resolvability of the interferograms. Higher frequency interferograms are well resolved without attenuation. The reconstructed A-scans therefore do not suffer from signal roll-off across the imaging range
The instantaneous line width characterizes how pure the laser mode is at one particular moment while it is swept through a range of individual wavelengths. Ideally, the tuning laser is emitting light at a single wavelength at any given moment. In reality, the emitted light is typically composed of photons of more than one wavelength spanning across a certain range that is characterized as instantaneous line width. Such wavelength impurity affects the interferogram similarly as finite pixel size of linear detector array in SD-OCT and causes SNR roll-off as well. However, with the recent advances in laser technology, the commercial tuning lasers have dramatically improved instantaneous line width [12]. Therefore, the interferogram fringes can be well-resolved across the entire imaging range. The SNR roll-off in SS-OCT systems enabled by the latest vertical-cavity surface emitting lasers (VCSEL) is virtually zero within the imaging range of up to 50 mm [13].
In addition, SS-OCT is able to operate in the 1 μm wavelength range. Besides low water absorption and minimal depth-dependent dispersion [14], 1 μm wavelength OCT is less susceptible to scattering in tissue such as cataract and hemorrhage [15]. It can also better penetrate the retina pigment epithelium (RPE) and visualize deeper structures in the choroid layer and sclera [16, 17]. The practical benefits of 1 μm wavelength SS-OCT can be appreciated by comparing retinal images at different depths acquired with an 800 nm wavelength SD-OCT system (Topcon 3D OCT-2000) and a 1 μm wavelength SS-OCT system (Topcon DRI-OCT Triton), as shown in Fig. 1.6.


Fig. 1.6
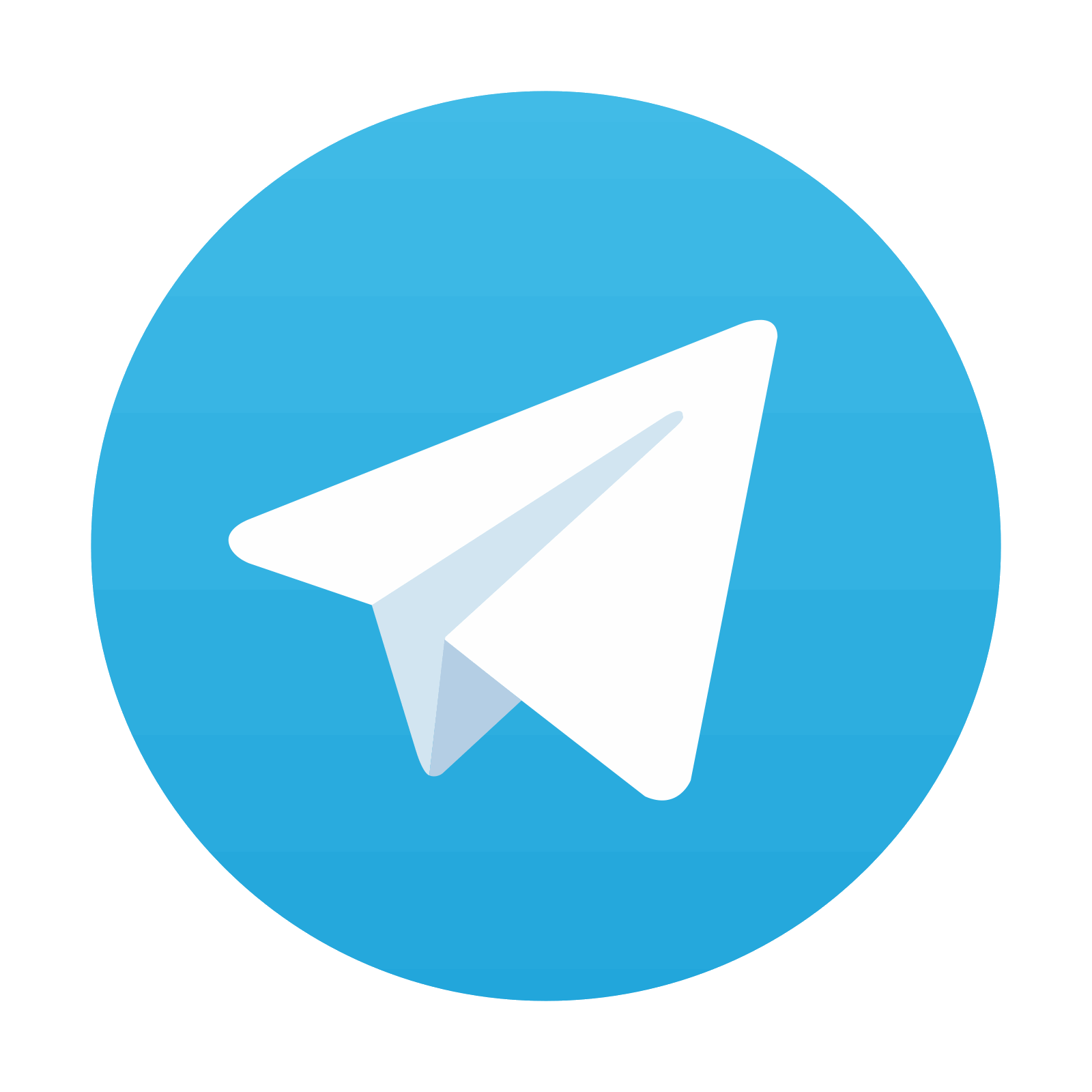
Retinal images at different depths acquired with 800 nm wavelength SD-OCT and 1 μm wavelength SS-OCT. 1 μm wavelength SS-OCT not only can visualize deeper structures in the choroid and sclera, but is also capable of obtaining high-quality images at any depth within the imaging range
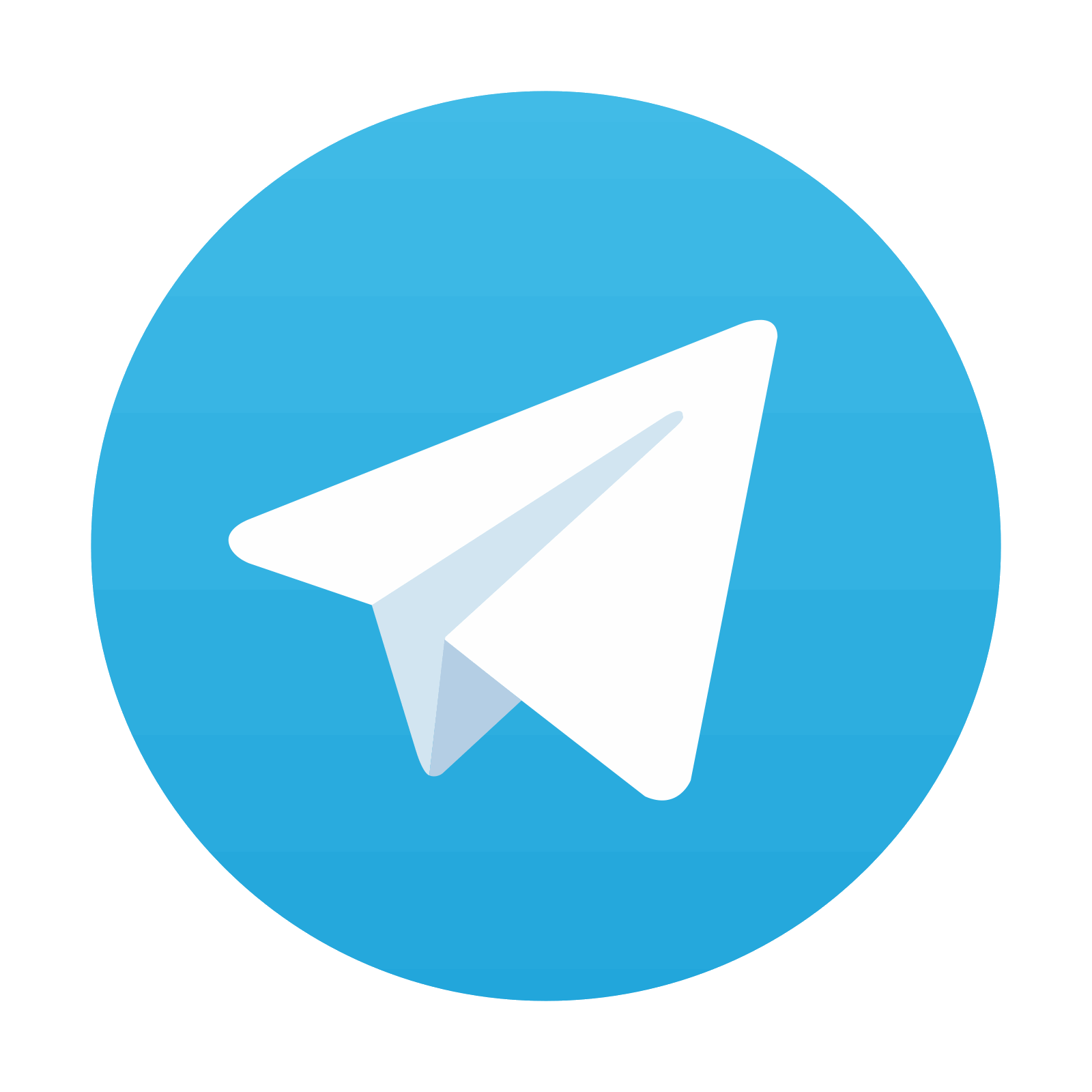
Stay updated, free articles. Join our Telegram channel
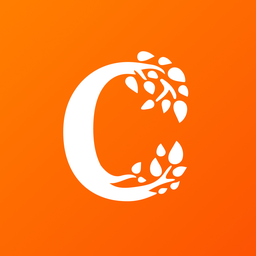
Full access? Get Clinical Tree
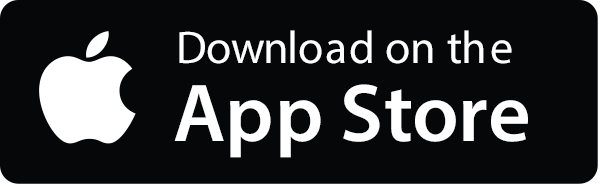
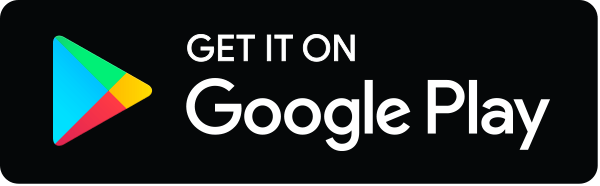
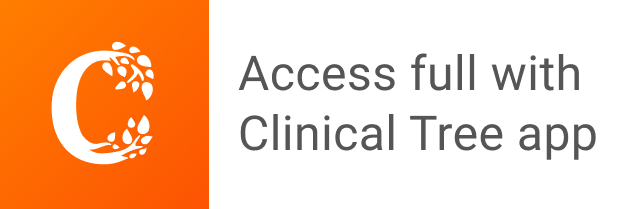