, Maneli Mozaffarieh1 and Hans Bebie2
(1)
Department of Ophthalmology, University of Basel, Basel, Switzerland
(2)
Institute for Theoretical Physics, University of Bern, Bern, Switzerland
Abstract
In interventions in the eye, various qualities of laser radiation play a role. Laser light can easily be focused and managed temporally; therefore, it permits enormous leeway in the choice of power and intensity. It also has a defined wavelength. The various tissues have different absorption spectra. This also influences the depth of the penetration of light. Depending on the type of laser settings (wavelength, pulse duration, energy, fluence, and irradiance), light interacts differently with tissue. Since we are not attempting to present a complete list but, instead, wish to explain the main mechanisms of light interactions with matter, we shall be limiting all explanations to the following categories, listed in Table 7.1:
In interventions in the eye, various qualities of laser radiation play a role. Laser light can easily be focused and managed temporally; therefore, it permits enormous leeway in the choice of power and intensity. It also has a defined wavelength. The various tissues have different absorption spectra. This also influences the depth of the penetration of light. Depending on the type of laser settings (wavelength, pulse duration, energy, fluence, and irradiance1), light interacts differently with tissue. Since we are not attempting to present a complete list but, instead, wish to explain the main mechanisms of light interactions with matter, we shall be limiting all explanations to the following categories, listed in Table 7.1:
Table 7.1
Categories of laser light interactions with ocular media. λ wavelength
Category | Physical processes | Typical application | Typical laser (λ) | Section |
---|---|---|---|---|
Photocoagulation | Heating, coagulation, evaporating, charring | Photocoagulation in diabetic retinopathy | Argon (514 nm) | Sect. 7.1 |
Photodisruption | Optical breakdown, cavitation, shock waves | Capsulotomy | Nd:YAG (1,064 nm) | Sect. 7.2 |
Photoablation | Splitting of molecules, removal of tissue | Refractive correction of the cornea | Excimer (193 nm) | Sect. 7.3 |
Photocutting | Optical breakdown, cavitation | Lamination of the cornea | Femto (1,040 nm) | Sect. 7.4 |
1.
Pure heating. This process is based on absorption by colored matter; its main application lies in coagulation of, e.g., the retina (Sect. 7.1).
2.
Optical breakdown inside transparent tissue, based on concentrating the pulse energy in very short pulse durations (Sect. 7.2). The associated high power densities lead to a kind of microexplosion with mechanical effects (forming a cavitation). One of its applications is capsulotomy.
3.
Removal of tissue at a surface. The process takes place via the splitting of molecules into small fragments that escape from the surface. One application is corneal refractive surgery (Sect. 7.3).
4.
Optical breakdown with extremely short pulse durations, also producing small vesicles but without thermal effects in the environment. Corneal incisions represent one application (Sect. 7.4). With extremely short pulse durations, the process is effective even with very low pulse energies.
The choice of wavelength – in the range of UV to IR – is partially dictated by the absorption properties of the tissue and its pigment(s) (details are provided in Sect. 2.8). For example, ultraviolet light from an excimer laser (ArF, 193 nm) is already absorbed within the superficial layers of the cornea and is, thus, well suited for tissue removal but unfeasible for applications in deeper layers. For other applications, the different absorption properties of pigments determine the optimum wavelength. Both the argon laser (514 nm) and the ruby laser (684 nm), each employed in its own way for applications in the ocular background, are optimal for very specific interventions since blood absorbs green-wavelength light (514 nm). Apart from the absorption spectra, there are also technical aspects: the Nd:YAG laser (1,064 nm) is suited for photodisruption due to its short and intense pulses. Figure 7.1 provides an overview of the wavelengths in various applications.
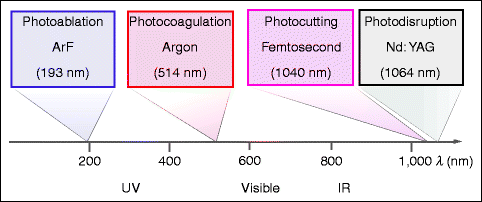
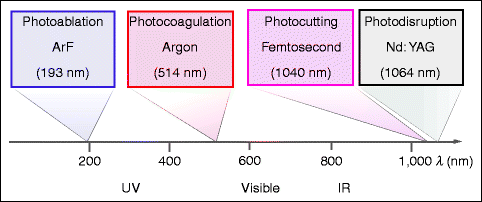
Fig. 7.1
Interventions at various wavelengths with typical lasers that are utilized (overview)
While pulse durations range over an enormous extent from 1 s down to extremely short pulses of 10−13 s (i.e., over 13 orders of magnitude), there is one parameter that differs far less in the various applications: the pulse energy per area at the focus, stated in J/cm2 (energy density, fluence). Typically, it lies in the range between 1 and 1,000 J/cm2 and, thus, varies by only about three orders of magnitude. The absorbed power per area in W/cm2 (irradiance) is, therefore, approximately indirectly proportional to the pulse duration, meaning that the choice of pulse duration more or less determines the power per area and, thus, essentially, the type of interaction between light and tissue. Figure 7.2 illustrates how the four principal modalities are clearly separated by pulse duration and irradiance. In comparison to the irradiance of sunlight (on the earth’s surface), these are extremely large values. The time units are listed in Table 7.2.
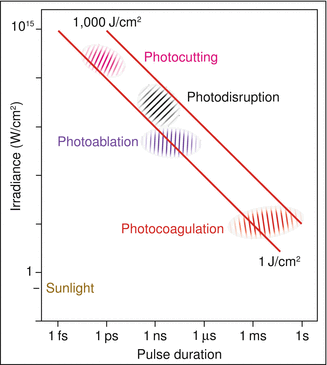
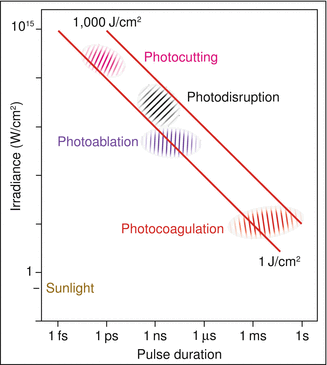
Fig. 7.2
Approximate pulse duration and irradiance of the various modalities. The precise values depend on the details of the application (e.g., the repetition frequency of the pulses). For comparison, the irradiance of intense sun radiation of the earth amounts to 0.1 W/cm2. Note that both scales are logarithmic
Table 7.2
The pulse duration in laser treatments of the eye extends over 13 powers of ten Here, the various time units are defined
1 fs | 1 ps | 1 ns | 1 μs | 1 ms | 1 s |
10−15 s | 10−12 s | 10−9 s | 10−6 s | 10−3 s | 100 s |
The purely physical relationship between pulse power per area (irradiance) at the focus and the pulse duration for a fixed pulse energy of 3 mJ is shown in Fig. 7.3. The irradiance is the determining variable for the type of interaction of light with matter (Fig. 7.2). It is not only determined by the pulse energy but also depends on the diameter of the focus.
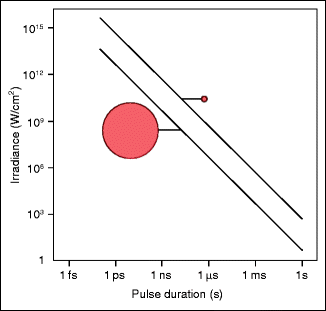
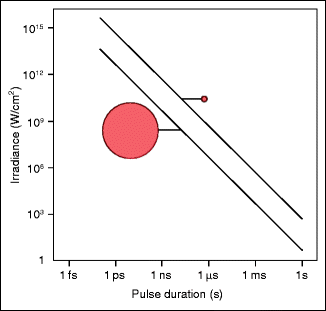
Fig. 7.3
The shorter the pulse duration is, the higher the irradiance will be. Irradiance is indirectly proportional to the pulse duration for a given pulse energy (3 mJ). The two curves refer to spot diameters of 30 and 300 μm. The smaller the spot diameter is, the larger the irradiance will be
7.1 Photocoagulation
The coagulation of the retinal tissue for the prophylactic treatment of diabetic retinopathy to prevent neovascularisation represents one of the oldest, most well-established interventions with light in the eye. The treatment effect arises via the heating of the retinal pigment epithelium and choroid through the absorption of light energy. Historically, the interventions with light began before the invention of the laser with the experiments of Meyer-Schwickerath2 carried out in Hamburg in 1949. He proceeded from the observation “that a progressive retinal detachment comes to stop at a retinal scar.” He was stimulated by his observation of a patient who had viewed a solar eclipse too long and thereby developed a central retinal burn (Fig. 7.4). At that time, the sun was the light source with the highest irradiance. Meyer-Schwickerath developed an apparatus that directed sun rays into the eye being treated: the sunlight coagulator. A heliostat (two slowly turning mirrors) compensated for solar movements. He was then involved in the development of the Zeiss light coagulator, which worked with a xenon high-pressure lamp and was marketed in 1957 (Fig. 7.5). Fresh impacts of retinal coagulation with a xenon lamp are depicted in Fig. 7.6. A broad discussion about the indication of photocoagulation started. Peripheral retinal lattice degenerations (Fig. 7.7) were treated by some physicians but not by others.
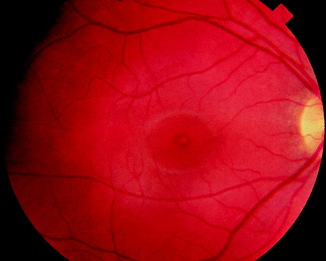
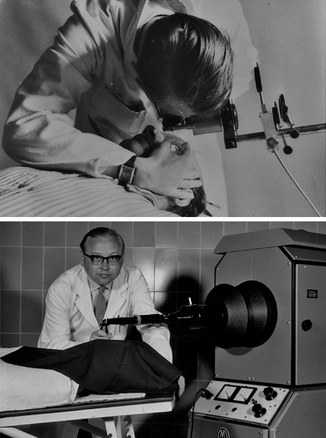
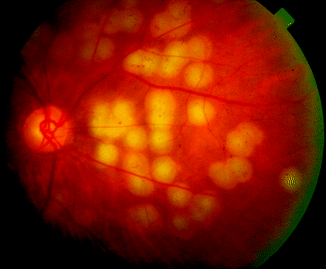
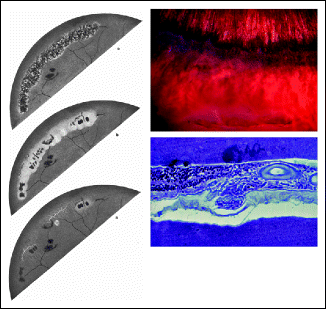
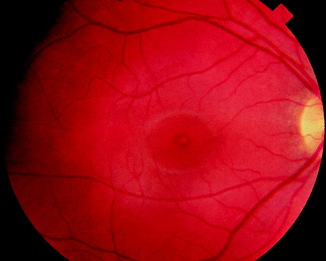
Fig. 7.4
Central retinal burn from viewing the sun
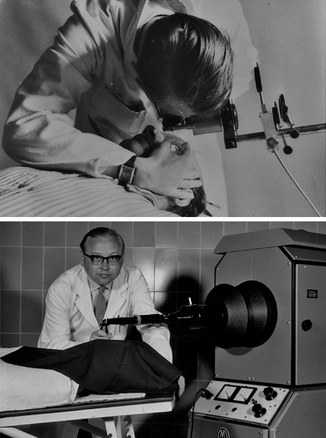
Fig. 7.5
Gerhard Meyer-Schwickerath coagulating the retina with sunlight (top) and a xenon coagulator (bottom) (Courtesy of R. Meyer-Schwickerath, Ahaus, Germany)
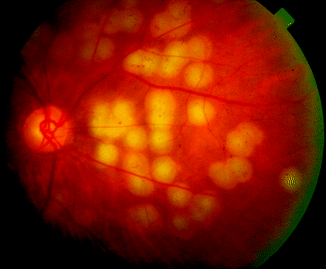
Fig. 7.6
Fresh retinal impacts following xenon light therapy
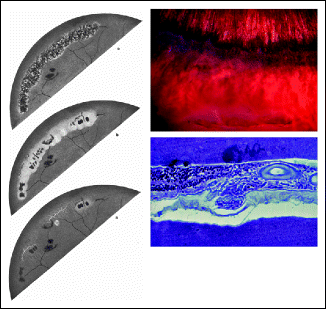
Fig. 7.7
Peripheral retinal lattice degeneration. Left: historical drawing (From Meyer-Schwickerath G (1954) Albrecht von Graefes Arch Ophthalmol, 156. With permission). Right: contemporary photo and histological picture (Courtesy of P. Meyer, University of Basel)
One year after the development of the first laser, Campbell (U.S.A.) introduced it to ophthalmology (ruby laser, 684 nm; see Sect. 3.4.1). The wavelength of the ruby laser is only weakly absorbed by blood, which makes it suitable for irradiating retinal tissue or choroidal alterations near blood vessels without the risk of hemorrhages. However, it turned out to be unsuitable for occluding blood vessels. The main absorbing structure on the fundus is the pigment epithelium. The heated pigment epithelium leads to a secondary heating and, thus, to retinal coagulation (Figs. 7.8 and 7.9).
< div class='tao-gold-member'>
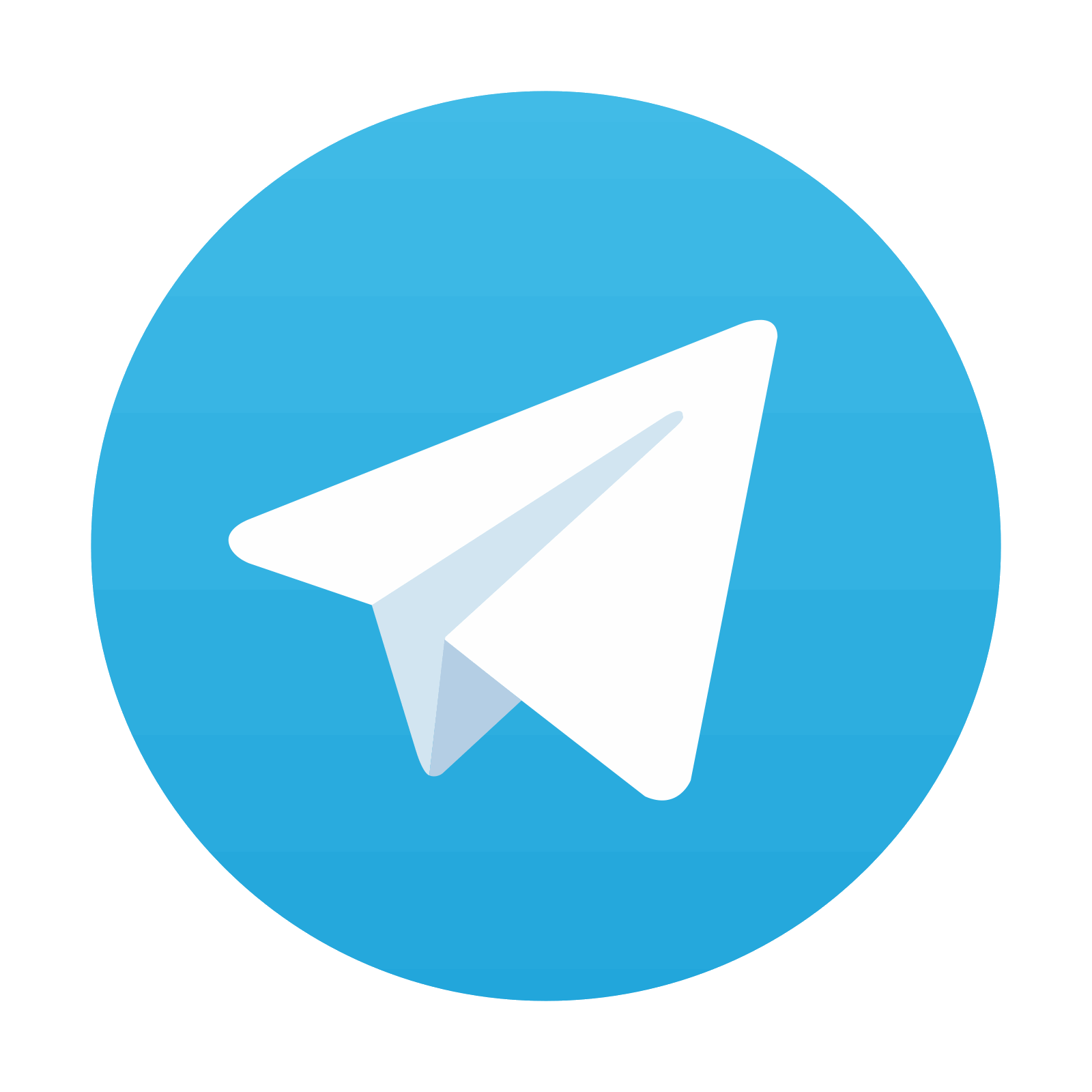
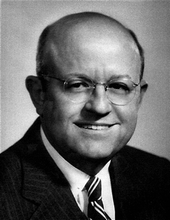
Only gold members can continue reading. Log In or Register a > to continue
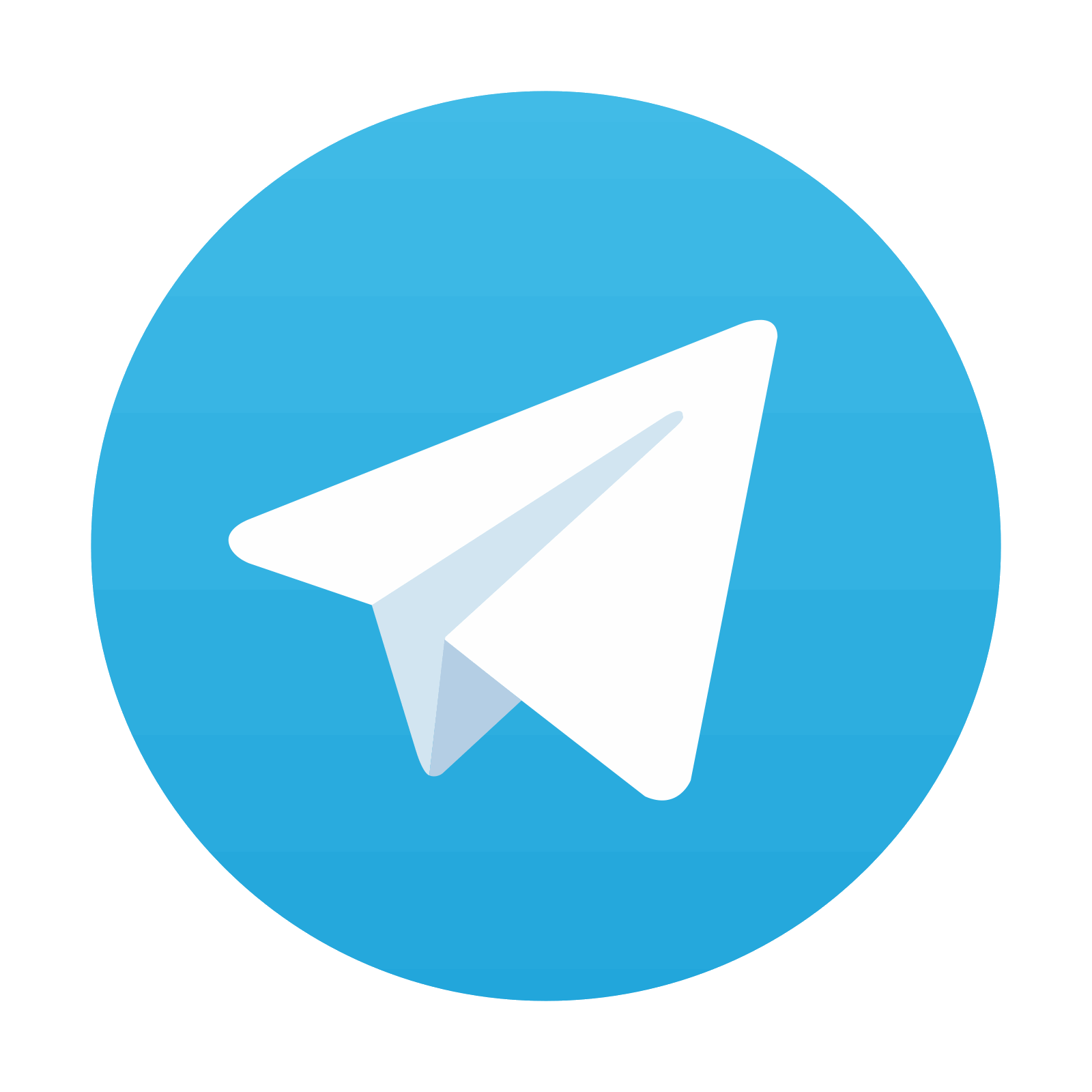
Stay updated, free articles. Join our Telegram channel
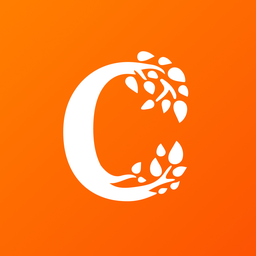
Full access? Get Clinical Tree
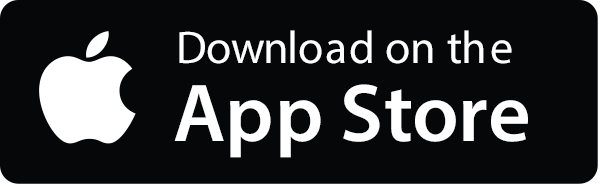
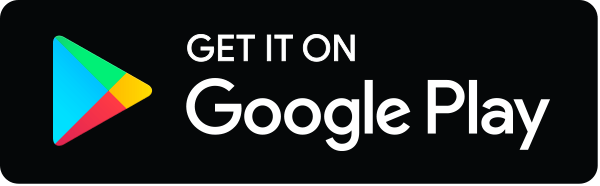