Lesions containing lipid
Dermoid/epidermoid cysts, lipomas, liposarcomas
Lesions containing mucusa
Dermoid/epidermoid cysts, mucoceles
Subacute blood
Hematic cysts, subperiosteal hematomas, blood cysts within lymphangiomas
Lesions containing melanin
Melanoma
The most useful views for the clinician on MRI of the orbit are precontrast T1 weighted and T2 weighted (often with fat suppression) and postcontrast T1 weighted with fat suppression. Three additional MRI techniques are worth mentioning, as they have practical implications for the orbital surgeon and neuro-ophthalmologist.


- 1.
When imaging a patient suspected of having demyelinating disease, T2-weighted fluid-attenuated inversion recovery (FLAIR) images are invaluable. The first signs of demyelination typically occur periventricularly, and in T2 weighting, the bright CSF makes this area difficult to interpret. FLAIR technique suppresses the signal from CSF and highlights areas of demyelination (Fig. 32.1).
- 2.
Diffusion-weighted imaging (DWI) and apparent diffusion coefficient (ADC) are routinely performed for brain, orbit, face, and neck MRI, and in spite of greater anatomic distortion and decreased resolution in the head and neck, these techniques help to more specifically characterize lesions. Briefly, DWI measures Brownian motion of water. In processes composed of tightly packed, small cells with a high nuclear-to-cytoplasmic ratio (e.g., lymphoma, retinoblastoma), diffusion is restricted, and a characteristic signal intensity is noted on DWI and ADC . Several studies have shown potential utility in distinguishing between orbital processes using this technique [8–12]. In addition, DWI is helpful in distinguishing abscess from other inflammatory processes; thick purulent material restricts on diffusion sequence.
- 3.
Three-dimensional constructive interference in steady state (3D CISS) is a fully refocused steady-state gradient-echo, T2 MRI sequence that is particularly useful for the evaluation of any structure surrounded by CSF, particularly the cranial nerves (Fig. 32.2) [13, 14]. Cavernous sinus imaging is also enhanced by this technique. 3D CISS is also of particular interest to skull base surgeons, since CSF rhinorrhea can be identified with these MR sequences [15]. Because of the physics of MRI, any image plane can be easily obtained, and for certain cranial nerves, oblique coronal and oblique sagittal views may be ideal. One major limitation of 3D CISS is its long acquisition time.

Fig. 32.1
T2 FLAIR of demyelination . (a) Axial, precontrast T1 MRI shows the largest lesion in the left frontal white matter. CSF is dark on T1. (b) On T2-weighted MRI, areas of increased signal suggestive of demyelination are noted. Note that CSF is hyperintense. (c) On T2 FLAIR imaging, the CSF is suppressed, and the areas of demyelination are obvious as increased intensity signal

Fig. 32.2
3D–CISS images of the oculomotor nerves. Axial, T2-weighted 3D–CISS MRI demonstrates the oculomotor nerves (arrows) traveling through the CSF-filled cistern
MRA approaches or equals the resolution of CTA in many academic centers but is a time-consuming technique. One advantage of MRA over CTA is that it may be performed (with less than ideal resolution) without contrast material; this is an important issue in patients who cannot tolerate contrast material because of medical comorbidities. Finally, MR venography (MRV) is exceedingly useful in evaluating patients for central venous sinus thrombosis. Any patient presenting with bilateral disc swelling suspicious for papilledema should undergo MRV as part of their work-up.
Pediatric Concerns with CT and MRI
Radiation exposure is inevitable with CT, and this is a major concern, especially in young patients who have a higher risk of cancer per dose of radiation as compared to adults. MRI is the modality of choice for evaluation of orbital and intracranial soft tissues and the visual pathway, but gadolinium use has risks in patients with renal disease and other morbidities. Because MRI requires exposure to a strong magnetic field, its use in patients with certain types of defibrillators and pacemakers or in patients harboring metallic foreign bodies may be contraindicated. MRI is also contraindicated as the initial imaging modality in evaluating trauma because of the possibility of an occult retained metallic foreign body.
With increased dependence on imaging techniques for the evaluation and management of orbital disease, it is important that clinicians also be familiar with the adverse effects of these modalities and ways to prevent them. In this section, we discuss the various pitfalls of using intravenous contrast agents for CT and MRI, with special emphasis on contrast-induced nephropathy and nephrogenic systemic fibrosis. We also discuss the risks of radiation exposure from CT and recommendations to minimize the radiation dose.
Contrast Agents
One of the major impacts of renal function in the evaluation of orbital pathology is the use of intravenous contrast agents for CT and MRI. The safety of intravenous contrast agents is highly dependent on renal function. Intravenous contrast (IVC) is the most common cause of iatrogenic acute kidney injury [16]. Contrast-induced nephrotoxicity (CIN) is uncommon in patients with normal kidney function, with an incidence of 1–2%. However, the incidence rises dramatically to as high as 25% in patients with chronic kidney disease [16]. CIN typically occurs within 3 days of contrast infusions with a peak serum creatinine at 3–5 days, usually returning to baseline in 7–10 days. Prevention of CIN mainly involves adequate precontrast hydration, either with intravenous isotonic saline or with unrestricted oral fluid intake [17]. Nonionic iodinated contrast material appears to be safer for the kidneys than ionic iodinated contrast material, at least when measured by creatinine clearance. As an aside, CIN from appropriately dosed gadolinium infusion is either nonexistent or exceedingly rare.
With regard to CT contrast agents, several issues must be stressed. First, because of the potential for seizure and vasculopathic complications with iodinated contrast, nonionic contrast agents are used in the vast majority of central nervous system imaging with CT, including the orbit [18]. Fatal reactions are rare, ranging between 1 in 20,000 and 40,000 examinations utilizing contrast material; of note, fatality rates are similar between high-osmolar ionic contrast and low-osmolar nonionic preparations. However, transient ischemic attacks and cerebrovascular infarctions during cerebral angiography occur much more frequently, at a rate of 1.6% in high-risk patients. Second, the seemingly age-old custom of inquiring about shellfish allergies as a possible harbinger of contrast allergy is unfounded as no reliable link has been identified. However, any history of a previous contrast-related reaction is important, as this increases the risk of subsequent reaction fivefold. Atopy (including asthma) also increases the risk of allergic reaction.
Intravenous corticosteroids have been recommended as a “premedication” for at-risk patients. It is important to note, however, that there is no unequivocal proof of short-term corticosteroid efficacy in preventing contrast-induced anaphylactoid reactions. Furthermore, adequate suppression of basophils and histamine production is typically delayed for 4–6 h after corticosteroid infusion, and such a delay may be untenable in emergency situations. One common algorithm in at-risk patients is an oral dosing of corticosteroids beginning at least 6 h prior to contrast infusion, with or without oral antihistamines. Low-osmolarity nonionic agents appear to have a lower incidence of allergic reaction. An excellent summary for management of a variety of contrast-induced reactions is available for download from the American College of Radiology (ACR) Manual on Contrast Media, version 9, 2013 [19].
Nephrogenic systemic fibrosis (NSF) is a scleroderma-like syndrome related to the infusion of gadolinium during MRI [20]. It is most frequently associated with renal failure, may be progressive, and may affect multiple organ systems. NSF is admittedly rare, and its incidence depends on the specific type of gadolinium compound used. As an example, gadodiamide has an NSF incidence of 1:2913 (0.03%), while gadopentetate dimeglumine has a much lower incidence of only 1:44,224 (0.002%) [20–22]. The overall incidence of NFS appears to be decreasing in the United States, likely because of the avoidance of higher-risk gadolinium preparations, more effective patient screening, and avoidance of use of gadolinium in high-risk patients, as well as more discriminant use of other MRI contrast materials.
NSF typically occurs in patients with the clinical triad of gadolinium infusion for MRI, renal failure (either chronic or acute), and a “pro-inflammatory state” (recent major surgery, endovascular injury, sepsis, malignancy, thrombosis, etc.) [23, 24]. In patients with significant renal failure, the incidence of NSF is estimated at about 4%, with 45% of affected patients suffering from severe disability and with a mortality of up to 31% [23, 24]. Of note, no effective therapy exists for treatment of NSF, and there is no evidence that pretreatment of patients with corticosteroids or pre−/post-gadolinium dialysis has any effect on the incidence and severity of NFS. Skin changes in NSF begin days to months after gadolinium infusion, with an average of 11.5–14 days [23–25].
Although NSF is rare, the severity and potential mortality of the syndrome requires cautious use of gadolinium in patients at higher risk for NSF [22, 23]. The US Food and Drug Administration (FDA) has published recommendations regarding gadolinium use, which are summarized in Table 32.2 [26].
Table 32.2
FDA recommendation regarding gadolinium use in MRI
Do not use gadolinium in: Patients with a GFR of <30 ml/min/1.73m3 Acute renal failure from hepatorenal syndrome Perioperative liver transplantation Unless diagnosis is essential with contrast-enhanced MRI |
Use gadolinium with caution in: Patients with a GFR of <60 ml/min/1.73m3 |
All patients undergoing contrast-enhanced MRI should be screened for kidney disease by history or laboratory evaluation: Screen all patients for acute kidney injury or other causes of decreased renal function Obtain GFR in all patients at risk for chronically reduced renal function (age >60 years, hypertension, diabetes mellitus, etc.) |
The American College of Radiology has recently noted in its Manual on Contrast Media (version 10.2) that dose-dependent gadolinium deposition has been noted within brain tissue in patients who underwent serial contrast-enhanced MRIs [19]. Deposition mainly occurred in the dentate nucleus and globus pallidus, but histopathologic examinations have also revealed gadolinium in the frontal lobe white matter and in the prefrontal cortex [27, 28]. Importantly, the deposition can occur in patients with an intact blood–brain barrier and no evidence of hepatic or renal disease and has been documented in children undergoing serial MRI with contrast. Thus far, the clinical significance of these findings is unknown, and no adverse effects have been noted, but the FDA is actively investigating this phenomenon. In the meantime, clinicians should be aware of this issue. Gadolinium infusion during MRI frequently provides critical imaging information and often needs to be used in initial, routine MRI [27]. However, in cases where serial MRI is indicated in a child (e.g., monitoring for glioma progression), the utility of a non-contrast MRI should be discussed with the neuroradiologist. Some experts also recommend the use of higher stability, macrocyclic gadolinium preparations, which have lower deposition rates [27, 28].
Radiation Exposure in CT
A significant amount of data has been published since 2001 on the subject of radiation exposure from computed tomography (CT) [29–32], resulting in a broad spectrum of interpretation by the media and in the literature [33, 34]. This, in turn, has led to appropriate concern among patients and the parents of young patients as to the potential cancer risk from CT. This section reviews the available data and summarizes the conclusions and recommendation of our radiology colleagues. Of note, most of the concerns regarding radiation effects of CT pertain to children (especially young children) as compared to adults [34].
First, it is critical to remember that CT is an extremely powerful diagnostic tool that is readily available and is appropriate to use when clinically justified, even in children [35]. As a blanket statement, the risk of potential future cancer from a single CT is far less than the diagnostic benefit, especially in emergency situations [36, 37]. CT requires the use of a certain amount of ionizing radiation – there is simply no way around the basic physics of the technology. However, the radiation dosage of a diagnostic CT can be adjusted to lower levels without compromising diagnostic quality – the ALARA concept (as low as reasonably achievable).
Until recently, the actual risk of future cancer from low-level radiation exposure was not definitively quantified by any large study [36]. The majority of published conclusions relied on extrapolation from atomic bomb victims where there was whole-body exposure or from mathematical models [34, 38]. However, since 2012, two major studies have been published which quantify CT radiation risk and have reached some sobering conclusions [39, 40].
The study of ionizing radiation effects on the body is complex and beyond the scope of this chapter. Briefly, however, radiation affects specific tissues in different ways. As an example, studies on atomic bomb survivors concluded that the lung is more sensitive to the carcinogenic effects of radiation exposure than the liver, skeletal muscle, or skin [29, 37]. Furthermore, the dose of radiation from a CT study that strikes the body (“entrance dose”) is not equivalent to the absorbed radiation by the body [39]. It is difficult to accurately quantify the risk of future malignancy from low-dose radiation exposure because low-dose radiation is a weak carcinogen [36, 39]. However, it is important to note that three expert panels, including the National Academy of Sciences, have concluded that there is a potential cancer risk even with low-level exposure and that this risk rises with increasing exposure [37]. In addition, the concept of “radiation effect” is critical in understanding the radiation risk from CT. Put simply, two types of radiation effects exist [34]. Deterministic effects are well known to the orbitologist and are typified by high-dose radiation to the orbit (or any anatomic structure) over a short period of time, usually for the treatment of malignancy, resulting in myriad side effects (e.g., keratopathy, retinopathy, optic neuropathy, etc.). Stochastic effects are secondary to low doses of ionizing radiation absorbed over a long period of time, as seen in serial imaging, be it CT, mammography, etc. Although the side effects from such stochastic radiation are not immediately obvious, they do accumulate over time and are definitely more of an issue in the pediatric, as compared to the adult population.
The risk of radiation exposure is significantly different in children than in adults for multiple reasons [37]. First, children are more sensitive to the effects of radiation exposure than adults because of active tissue growth and development, with an estimated tenfold overall increased neoplastic potential [30]. Second, because of their age and statistically longer life span, children have more time in the ensuing decades to manifest any ill effects of radiation exposure; put simply, it is far more likely to see a radiation effect that takes 40 years to manifest in someone who is 10 years old than in someone who is 50 years old although, until recently, the magnitude of this risk from low-level exposure was difficult to accurately quantify. Third, unless the baseline radiation exposure parameters are adjusted for children, an equivalent dose has a higher effect on the smaller cross-sectional anatomic area of a child than of an adult; even with such adjustment, the final radiation dose effect to a child may be up to 50% higher than in an adult [41, 42]. Fourth and perhaps most pertinent to the reader, the pediatric brain is especially sensitive to radiation effects [39], and at least a portion of the brain is unavoidably imaged during an orbital study. Finally, the ubiquity of CT in medicine and expanding indications for CT has led to ever-increasing utilization.
The use of diagnostic CT in the United States has risen by more than 20-fold, from 2 million studies annually in 1980 to almost 80 million in 2009 [31, 32]. Seven million CT studies are performed on children annually in the United States, and this number increases an average of 10% per year. Of note, the majority of brain CTs performed in children is for head trauma [30, 40, 43–45]. CT also accounts for a disproportionately high amount of total radiation exposure in medicine. A study from one academic radiology department published by Mettler and colleagues in 2000 concluded that although CT accounted for only 11% of procedures using ionizing radiation , 67% of all ionizing radiation was attributable to this modality [45].
Perhaps most importantly, CT of one organ system can be performed using a variety of techniques with a wide range of radiation exposure. This concept is extremely important to keep in mind for pediatric CT. As noted in a review by Brody and Frush, increasing the dose of radiation in an effort to improve image (and presumably diagnostic) quality has its limits, and after a certain threshold, additional radiation simply has no effect on improved diagnostic accuracy [37]. Therefore, lower doses of ionizing radiation in pediatric CT are preferable and do not necessarily compromise the quality of the study. The ALARA concept has markedly reduced radiation exposure in children from CT studies, by 50–90% since about 2001, and these techniques continue to undergo refinement [30, 37, 40, 46].
The results of two recent large studies on radiation exposure from CT in children are worth summarizing in detail. Pearce and colleagues analyzed a national health-care database in the United Kingdom and identified just over 175,000 pediatric patients that met the inclusion criteria of the study [39]. Of note, of 283,919 CT scans performed, the majority (64%) were of the head, an area of particular importance to the reader. The authors of the study estimated that the average total dose from one brain CT in a child ranged between 28 and 44 mGy depending on age and gender (Table 32.3) [39]. The rates of leukemia and brain tumors in patients undergoing CT were analyzed based on dose. A significantly increased risk of both maladies was found in patients who received certain threshold cumulative radiation doses. Specifically, the relative risk (RR) of leukemia in patients with a cumulative dose of at least 30 mGy (mean 51.13 mGy) was 3.18 compared to those children who received less than 5 mGy. With regard to brain tumors, the RR was 3.32 in patients with a cumulative dose of 50 mGy (mean 104.16 mGy). The authors estimated that for children younger than 15 years, these threshold cumulative doses amounted to five to ten head CTs for a 50 mGy bone marrow dose and two to three head CTs for a 60 mGy brain dose. Put another way, the authors concluded that one head CT before the age of 10 years would result in one excess case of leukemia and one excess brain tumor per 10,000 patients. Of note, multiple studies have concluded that younger children, especially those <5 years of age, are particularly sensitive to ionizing radiation [39, 40]. Admirably, Pearce et al. also pointed out that, first, pediatric leukemia and brain tumors are relatively rare events and that tripling this risk from CT radiation exposure still makes these entities rare events and, second, the increased lifetime cancer risk from CT is “very small” compared with the natural occurrence of malignancy in the general population which may make the benefits of CT more important “providing it is clinically justified.” Herein lies the crux of the discussion: while CT is a powerful and lifesaving technology, with a now-proven underlying cancer risk, it therefore should be used only when appropriate and then very judiciously.
Table 32.3
Estimated radiation doses from one brain CT utilizing estimated standard CT dosages after 2001
Males | Females | |||
---|---|---|---|---|
Age atbrain CT(years) | Braindose(mGy) | Red bonemarrowdose (mGy) | Braindose(mGy) | Red bonemarrowdose (mGy) |
0 | 28 | 8 | 28 | 8 |
5 | 28 | 9 | 28 | 9 |
10 | 35 | 6 | 35 | 6 |
15 | 43 | 4 | 44 | 6 |
20 | 35 | 2 | 42 | 2 |
In the second large study, Mathews and colleagues compared 680,211 pediatric patients who underwent CT with 10,939,680 who did not [40]. Mathews et al.’s study echoed the findings of Pearce et al. regarding subsequent leukemia development, providing independent confirmation of the previous study’s findings; a cumulative dose of 30 mGy resulted in a relative risk of 3.18. Brain CT was associated with an excess incidence rate for brain cancer of 2.97/100,000 person-years. Put another way, the authors estimated 1 excess brain malignancy per 4000 brain CTs. As already noted, the authors also confirmed that children <5 years of age had the highest relative risk. However, even older children and adolescents showed an increased cancer risk following CT. Serial CT also increased the risk, presumably due to increased cumulative radiation dosages. Of particular interest to the reader, facial CT was associated with only a borderline increased RR of subsequent malignancy (IRR = 1.14). Since an orbital study is essentially a subseries of a standard maxillofacial protocol, it is reasonable to extrapolate this facial CT finding for orbital scans. In other words, based on the findings of Mathews et al., an orbital series should have a lower risk for subsequent brain or hematopoietic tumor development than a standard brain CT. However, this statement must be tempered by the varying protocols used at different health-care facilities, some of which may extend a standard orbital series more cephalad, thereby exposing more of the brain to radiation, as well as the fact that in many (if not most) orbital injury cases, the head is scanned along with the orbits as a precaution.
Mathews and colleagues also brought up some important limitations to their study (which do not change, however, the prevailing opinion among radiologists that even low-dose radiation exposure increases cancer risk, albeit by a very small amount). First, as already noted, the authors point out that CT dosing protocols, especially for pediatric scans, have undergone a profound change since 2001 and that the current cancer risk from CT radiation may be smaller than calculated from older data. Second, the possibility of reverse causation may explain some of the increased brain tumor risk seen from head CT. In other words, symptomatology may have prompted initial brain imaging, which failed to diagnose an early, small lesion that went on to grow until the diagnosis was made several years later; thus, the undiagnosed tumor prompted the initial CT, and therefore the CT did not cause the tumor. Of note, the authors still found an increased solid and hematopoietic cancer risk following CT exposure, even when all brain tumors were excluded from analysis.
What, then, is the role of CT in pediatric orbital disease? First, as already stated, it is important to recognize that CT remains a valid and powerful tool in the pediatric population. In cases of head and orbital trauma, CT remains the imaging modality of choice because of its ready availability, rapid acquisition time, excellent resolution of bony and soft tissue orbital anatomy, and reasonable resolution of brain anatomy, especially in cases of acute intracranial hemorrhage. That said, it is also important to remember that a pediatric brain CT carries an increased risk of brain malignancy when compared to facial (i.e., orbital) CT based on the results of Mathews et al.’s study and a brain CT should not be a knee-jerk response to every case of pediatric orbital trauma.
The authors recommend the following guidelines for the judicious and appropriate use of orbital CT, much of it gleaned from the recommendations of the American College of Radiology :
- 1.
The right scan for the right pathology. In general, CT is excellent for imaging bone and therefore is useful in the evaluation of trauma and orbital infection (which is often secondary to adjacent paranasal sinus disease). CT is also helpful in the diagnosis of metallic and wooden foreign bodies [47]. Unless indicated, avoid including a brain CT with the orbital study. Consider MRI in nonurgent orbital pathology.
- 2.
Avoid plain X-rays. There are few indications for “screening” plain films of the face (see Table 32.4). On occasion, a plain X-ray is necessary to rule out occult metal prior to MRI. Otherwise, plain films should be avoided; they simply do not provide adequate detail of orbital or periocular pathology and still expose the patient to radiation.
- 3.
Order the right scan the first time. An axial imaging study of the brain is not equivalent to a dedicated orbital and facial study with axial and coronal views. Sometimes both the central nervous system and the orbit require imaging, but very often, imaging of the orbit suffices. In one study, the estimated effective dose from a head CT in a 5-year-old child was 4mSV, equal to about 200 chest X-rays [37]. On occasion, the clinical findings may obviate the need for CT. As an admittedly controversial example, one could argue that routine CT imaging in a pediatric white-eyed blowout fracture (WEBOF) is of little use because of the pathognomonic clinical features and predictable intraoperative findings; that said, at present, preoperative CT imaging of a suspected WEBOF remains the standard of care.
- 4.
Communicate. Discuss with the radiologist or emergency room physician the specific area of concern, stressing the need for axial and coronal views limited to the orbit, unless brain imaging is indicated.
- 5.
Insist on seeing the actual scan (and not simply the report) if the patient is being referred from another institution. In more routine cases, a delay of several days while the mislaid compact disc of the scan is being mailed is of little consequence. However, in an urgent situation (WEBOF, orbital abscess, etc.), absence of the actual images may force the physician to repeat the study, resulting in more radiation exposure for the child. If possible, make every attempt to obtain a digital copy of the original scan in a timely fashion to avoid reimaging.
- 6.
Make sure that your imaging facility has specific pediatric protocols with decreased radiation exposure using the ALARA concept. All facilities accredited by the American College of Radiology must have pediatric protocols in place [37].
- 7.
When possible, maximize diagnostic accuracy by having the study interpreted by a pediatric radiologist or neuroradiologist. Discuss any specific clinical suspicions (WEBOF, occult wooden foreign body, etc.) with the radiologist prior to the study to maximize CT technique.
- 8.
When possible, use single-pass technology. Newer multidetector CTs, such as CTA, are extremely useful in certain clinical situations but are usually unnecessary in typical trauma or infection cases [46].
- 9.
Consider other imaging modalities (MRI, ultrasound), especially if serial imaging is needed. When possible, use MR angiography rather than CT angiography in children.
- 10.
Discuss with the patient’s parents the rationale for the use of CT in their particular clinical situation, the necessity of ionizing radiation in CT, and the fact that radiation dosages will be age and size adjusted. In one study, a majority of parents were unaware that CT exposed their child to ionizing radiation [48]. As importantly, this study also found that providing information about radiation exposure in CT did not change the parents’ decision to proceed with imaging.
Table 32.4
Orbital imaging options in a child presenting with exophthalmos and their relative radiation risk
Radiologic procedure | Rating | Comments | Relativeradiation level |
---|---|---|---|
MRI head and/or orbit without/with contrast | 8 | See sections on nephrogenic systemic fibrosis and gadolinium deposition in brain | 0 |
MRI head and/or orbit without contrast | 7 | Lack of contrast limits the detail of normal anatomy and pathology | 0 |
MRA head and neck without/with contrast | 4 | See sections on nephrogenic systemic fibrosis and gadolinium deposition in brain | 0 |
CT head and/or orbit with contrast | 6 | See sections on radiation exposureand contrast-induced nephrotoxicity | ![]() ![]() ![]() |
CT head and/or orbit without contrast | 5 | Lack of contrast limits the detail of normal anatomy and pathology | ![]() ![]() ![]() |
CTA head and neck | 2 | CTA results in significant radiation exposure. Use MRA when possible | ![]() ![]() ![]() ![]() |
Plain X-ray of orbit | 1 | Although radiation exposure is low, plain X-ray of the orbit has very low diagnostic value. Usually limited to ruling out occult metallic foreign body prior to MRI in suspected cases | ![]() |
Table 32.4 from the American College of Radiology provides an excellent summary of various orbital imaging options and their relative radiation risk for a child presenting with exophthalmos [49]. Note that MRA carries a safer rating than CTA and does not require ionizing radiation . It is also important to note that a plain X-ray of the orbit carries the worst rating because it provides essentially no clinical information despite exposure to (albeit low) doses of ionizing radiation .
Developmental Anomalies
Imaging modalities play a major role in the evaluation of a child with a developmental ocular or orbital lesion [2]. While there often is external evidence of an abnormality, the full extent of the malformation can be appreciated only on multiplanar images. Various orbital deformities, bony defects, or calcifications/ossifications are best shown with CT, but the morphology of soft tissue derangements is best demonstrated with MR, including associated intracranial abnormalities.
Abnormal Globe
Imaging is helpful in delineating and characterizing globe abnormalities, which include anophthalmia (Fig. 32.3), microphthalmia (Figs. 32.4 and 32.5), macrophthalmia (Fig. 32.6), colobomas (Figs. 32.7 and 32.8), buphthalmos, and staphylomas. In ophthalmology, colobomas refer to defects in ocular structures that result from incomplete closure of the fetal (embryonic) fissure. Colobomas are common developmental malformations and usually are bilateral [50]. In cases of coloboma, the optic nerve head may be small and slit-like (Fig. 32.7), or there may be a large, funnel-shaped staphylomatous excavation with the optic disc at the apex of the defect. The latter type of coloboma is also referred to as morning glory syndrome. Large colobomas are easily detected (Fig. 32.8), but even small defects in the optic nerve heads can be shown on images (Fig. 32.7) when a high-resolution, thin-section T2 sequence is utilized or with a particularly specific sequence such as HASTE (half-Fourier acquisition single-shot turbo spin echo) which shows excellent contrast between fluid-filled and soft tissue structures (Fig. 32.9). Microphthalmos with cyst represents a severe colobomatous malformation when the inner layer of retina proliferates and herniates through the enclosed fetal fissure to form one or more cysts, which may be small (Fig. 32.10) or very large (Fig. 32.11).











Fig. 32.3
Fetal MRI of anophthalmia . (a) A T2 image (fetal axial) of a 26-week gestation fetus with hydrancephaly. Note that the cranium is filled with CSF. The right globe is essentially an extension of the CSF communicating via a widened nerve sheath (left arrow) with absence of the optic nerve. There is no lens. A small cystic projection is present in the left orbit with no visible globe (right arrow). (b) Fetal coronal T2 image shows the abnormal right globe (arrow), with left anophthalmia

Fig. 32.4
Bilateral microphthalmia with chronic retinal detachment in a 31/2-year-old autistic child. (a) Axial proton density-weighted MR image reveals bilaterally small globes with retinal detachment (straight arrows). The curved arrow points to isointense subretinal fluid. (b) Axial T2-weighted image reveals marked hypointensity along the detached retina due to calcification. The calcification was identified on computed tomography (not shown here). Note that the isointense subretinal fluid in the right eye on proton density has become hyperintense on T2 imaging

Fig. 32.5
Fetal MRI of microphthalmos in a 25-week gestation fetus with a hypoplastic right heart and left cleft palate. (a) Fetal axial T2 image shows right microphthalmia with a small posterior coloboma and a deformed, malpositioned lens. A thin stalk (arrow) projecting from the lens to the coloboma most likely represents persistent hyperplastic primary vitreous. (b) Sagittal fetal T2 image again demonstrated the deformed, small globe with a distorted lens

Fig. 32.6
Congenital glaucoma in a 3-month-old child. (a) Axial CT reveals a markedly enlarged left globe with expanded anterior chamber. (b) A three-dimensional CT soft tissue reconstruction shows well the enlarged left globe. (c) Three-dimensional CT bone reconstruction of the orbits reveals marked asymmetry, with an enlarged left orbit


Fig. 32.7
Bilateral optic nerve head colobomas in two infants. (a) Axial T2-weighted HASTE image shows slit-like defects (arrows) in the optic nerve head regions. (b) Coronal HASTE image obtained through the posterior portions of the globes reveals the colobomas (arrows). HASTE imaging is sensitive to the presence of fluid, and even though it does not reveal in detail other portions of the orbit, it is useful whenever there is a question of a fluid-containing abnormality, such as a cleft, cyst, or fistula. Each image takes 1.2 s, and, therefore, HASTE imaging often can be performed without sedation. (c) Axial conventional T2-weighted MR scan in another infant shows a coloboma (arrows) with an abnormally widened right optic nerve adjacent to the coloboma. (d) Axial T2 scan slightly inferior to that shown in (c) reveals a smaller coloboma (arrows) on the left. The left optic nerve is also abnormally widened just posterior to the coloboma. HASTE half-Fourier acquisition single-shot turbo spin echo

Fig. 32.8
Left morning glory coloboma in a 2-year-old girl. Axial CT scan reveals left microphthalmia and a large posterior coloboma (arrows), having a configuration reminiscent of the morning glory flower

Fig. 32.9
Fetal HASTE MRI of bilateral colobomas in a 22-week gestation fetus. (a) Sagittal maternal and axial fetal image. (b) On close-up, the axial T2 HASTE image reveals a large right coloboma (arrow). (c) In this image, a subtle left coloboma is also evident (right arrow)

Fig. 32.10
Microphthalmia with two cysts in a 3-week-old boy. (a) Axial T1-weighted MR shows a very small globe with deformed lens (white arrow) and an irregular cyst (black arrows) lateral to the globe. (b) Axial T2-weighted image better differentiates the small globe from the laterally located cyst. Note that the lens has become very hypointense. (c) Sagittal MRI reveals another cyst (arrows) inferior to the abnormal globe

Fig. 32.11
Left microphthalmos with a large cyst. Axial CT shows a very large cyst (white arrows) that expands the bony orbit and a very small malformed globe (black arrow) with an irregular denser lens (Courtesy of Richard Boyer, MD, Primary Children’s Medical Center, Salt Lake City, Utah)
Buphthalmos
Buphthalmos , or an enlarged eye (“ox eye”), is most commonly seen in primary congenital or early-onset infantile glaucoma; there is also an association with neurofibromatosis type 1, due to secondary glaucoma from neurofibromas. In addition, intraocular pathology, including tumor, can also lead to globe enlargement. In primary congenital glaucoma, there is resistance to normal outflow of aqueous humor due to an abnormally developed trabecular meshwork. This results in increased intraocular pressure, which causes enlargement of the globe and thinning of the sclera [51]. CT or MR demonstrates not only the macrophthalmia with an enlarged anterior chamber and flattened lens but also its effect on the bony orbit, which may have up to 15% increased volume (Fig. 32.6).
Optic Nerve Hypoplasia
Minimal symmetrical hypoplasia of the optic nerves may be difficult to detect clinically as well as on images. However, if the optic hypoplasia is unilateral, making comparison to the normal side possible, or if the hypoplasia is moderate to severe, then it can be detected on images. In such cases, it is important to obtain thin, contiguous sections and to look at the nerves in more than one plane to avoid making an error due to volume averaging. Optic nerve hypoplasia may be an isolated anomaly or may be associated with other abnormalities, as in the case of septo-optic dysplasia (Fig. 32.12) [52]. Coronal T2-weighted MRI is usually the best technique for evaluation of optic nerve size.


Fig. 32.12
Septo-optic dysplasia in a 2-month-old girl. (a) Axial T2-weighted MRI shows markedly narrowed optic canals (arrows) reflecting optic nerve hypoplasia. (b) Coronal T2-weighted image shows the typical absence of septum pellucidum (arrow points to where the septum pellucidum should be located). Normally this section should show the chiasm in the suprasellar region; it is not identified in this case because it is so hypoplastic. (c) Axial T1-weighted image at level of suprasellar cistern shows a very thin chiasm (arrow)
Cryptophthalmos
In cryptophthalmos , there is failure of development of lid folds, and as a result, the eyes are hidden by continuous overlying skin (Fig. 32.13) [53, 54]. The underlying globes typically are abnormal, having large anterior colobomas with absence of lenses or abnormal anterior chambers (Fig. 32.13). Cryptophthalmos is part of a syndrome that includes anomalies of extremities with craniofacial and urogenital abnormalities.


Fig. 32.13
Cryptophthalmos in a newborn boy with Fraser syndrome . (a) A clinical photograph of a different patient with cryptophthalmia reveals complete absence of lids. (b) Coronal CT shows the abnormal globes, disorganized anterior segments with calcifications (arrows), as well as a midline facial abnormality with abnormally wide nasal cavities and a cleft palate. (c) A 2-mm-thick axial CT section reveals continuous skin over the globes, which are abnormal and have expanded, partially calcified (arrows) anterior cysts (colobomas) instead of anterior chambers. The lenses are absent. (d) Axial T1-weighted MRI shows continuous skin with some underlying subcutaneous fat (arrows) over each globe. Again demonstrated are the abnormal globes with expanded anterior cysts and absence of lenses. The calcifications that were noted on computed tomography are not visualized on MRI. (e) Axial T2-weighted MRI shows markedly abnormal globes. A thin septum (arrows) can be identified separating the anterior cysts from the globes. The cysts have slightly greater intensity than the globes
Dermoid and Epidermoid Cysts
Dermoid and epidermoid cysts are the most frequent developmental cysts to occur in the orbit and represent choristomas, which are malformations of tissue that normally are not found at a given anatomic site. These cysts result from sequestration of ectoderm in the wall of the orbit or within the lids by advancing midfacial and frontal waves of neural crest during embryogenesis and typically occur either superolaterally (frontozygomatic suture) or superomedially (frontoethmoidal suture). Dermoid cysts, which are more common than epidermoid cysts, are lined by keratinizing squamous epithelium and contain dermal appendages (sebaceous glands, hair follicles) and keratinaceous debris and fatty substances. Epidermoid cysts are lined by epidermis without adnexal structures and contain desquamated keratin. The slow enlargement of the cysts causes pressure erosion of the adjacent bone, resulting in flattening (Fig. 32.14), thinning, and scalloping of the bone (Fig. 32.15). Occasionally such a cyst may break through the bone and displace dura mater. These developmental cysts have well-defined margins unless they have ruptured and caused an inflammatory reaction (Fig. 32.16). Also, the cysts may be associated with tracts that extend into adjacent structures (e.g., temporalis fossa, cranial vault, paranasal sinuses).





Fig. 32.14
Paraorbital, preseptal dermoid cyst in an infant boy. (a) Axial CT shows a small, well-defined, subcutaneous mass (arrows) with a denser capsule. The dermoid causes slight flattening of the bone on which it lies. (b) Coronal CT shows the dermoid (arrows) with a denser capsule. The black arrow indicates the flattened bone, which is in contact with the dermoid cyst

Fig. 32.15
An inclusion cyst in a 2-week-old girl either representing a dermoid or an epidermoid cyst . (a) Axial T1-weighted MRI shows an oval-shaped extraconal mass (white arrow), which displaces the globe and is associated with thickening and deformity of the lateral orbital wall (open arrow). (b) Axial T2-weighted MR shows the hyperintense oval-shaped mass and the scalloping of the lateral orbital wall. The signal characteristics in this case indicate that the cyst contains little fat or viscous mucus


Fig. 32.16
Ruptured dermoid cyst . (a) Coronal T1-weighted MRI shows a right extraconal mass (arrows) that has an irregular margin due to inflammatory reaction. (b) Axial T1-weighted image shows a slightly heterogeneous oval extraconal mass (arrow) with an irregular margin. The region of slightly increased intensity within the mass is consistent with lipid. (c, d) Proton density and T2 images reveal the dermoid to be of heterogeneous increased intensity and show well the focal bone scalloping (arrow)
The density on CT or the intensity on MRI of dermoid or epidermoid cysts ranges from that of cerebrospinal fluid to that of fat, and the lesions may be heterogeneous due to mixture of fat and other desquamated debris (Fig. 32.17) [55]. The heterogeneity of the signal coupled with pockets of hyperintensity on precontrast T1-weighted images helps to distinguish dermoid/epidermoid cysts from other orbital pathologies. Only a few orbital processes manifest this hyperintensity on precontrast T1-weighted images, and these exceptions are worth remembering, as they narrow the differential diagnosis (Table 32.4). Occasionally, there may be calcification in the capsule of a dermoid/epidermoid cyst. As just noted, identification of the lipid/protein content leads to the correct diagnosis, but most cysts tend to be isointense to extraocular muscle, and the diagnosis is often based on location and morphology. Only the cyst capsule shows enhancement with contrast. On DWI, most cysts show restricted diffusion – an important sign that reinforces the diagnosis of dermoid/epidermoid cyst (Fig. 32.18).



Fig. 32.17
Large epidermoid cyst of the right orbit. (a) Axial, precontrast T1-weighted MRI shows a spherical, well-defined mass. Note the heterogeneity in signal. The hyperintense signal posteriorly (arrow) represents the lipid component of the cyst. (b) Coronal, precontrast T1-weighted image also shows the lipid component (arrow). (c) Axial T2–weighted image. (d) Postcontrast, T1-weighted MRI with fat suppression reveals enhancement anteriorly. The fat signal in the posterior portion of the cyst is suppressed (arrow). Note that the intralesional fat mirrors the signal intensity and behavior of the normal intraconal orbital fat

Fig. 32.18
DWI of a dermoid cyst . (a) Axial, precontrast T1-weighted MRI of a dermoid cyst (arrow) near the left frontoethmoidal suture. Note that in this case, the lesion is hypointense but brighter than vitreous. This is likely because of the lack of intralesional lipid; the cyst is mostly made up of mucus. (b) On axial T2 weighting, the cyst (arrow) is hypointense to vitreous, but not completely dark. (c) Axial DWI shows the bright signal of restricted diffusion (arrow), again likely due to thick mucus within the lesion. (d) Axial diffusion tensor imaging. (e) Axial ADC. The pattern seen on images (c–e) (arrows) is consistent with restricted diffusion due to the viscous contents of the dermoid cyst
A developmental cyst should be differentiated from dacryocystocele (Fig. 32.19), which forms due to obstruction at the intranasal opening of nasolacrimal duct (valve of Hasner) and may be seen in a newborn. In such a case, one can identify a markedly dilated nasolacrimal duct (Fig. 32.19b); furthermore, the inferomedial location of a dacryocystocele is an uncommon site for dermoid/epidermoid cyst formation.


Fig. 32.19
Dacryocystocele due to obstruction of nasolacrimal duct in a newborn boy. (a) Axial CT shows a cystic mass (arrows) medial to the left globe. (b) Axial CT of nasal cavity reveals a dilated nasolacrimal duct (wider arrow). The black, narrow arrow indicates the normal duct
Orbital Teratoma
Orbital teratomas are choristomas (tissue at the wrong anatomic location) that are composed of tissue from all three germ cell layers – ectoderm, mesoderm, and endoderm – and are usually benign. These congenital tumors typically contain disorganized tissues but, rarely, may have fetal features. It is speculated that lack of normal response of tissue to inducers results in teratoma formation [56, 57]. Orbital teratomas generally present at birth with marked proptosis and then continue to grow rapidly [58]; they can also be demonstrated on fetal MRI. CT and MR demonstrate the teratomas as large multicystic masses that cause prominent expansion of the bony orbit (Fig. 32.20) [2]. Imaging studies are important in delineating teratomas and identifying their vascularity, determining whether there is an intracranial component and differentiating the teratomas from microphthalmos with a cyst, dermoid cyst, meningoencephalocele, and hemangioma or venolymphatic malformations located deep within the orbit.


Fig. 32.20
Cranio-orbital teratoma . (a) Clinical photograph of a newborn with marked right exophthalmos. (b) Sagittal T2-weighted MRI reveals a heterogeneous mass filling the right orbit and extending through the skull base intracranially. (c) Axial T2-weighted MRI. (d) Axial, postcontrast T1-weighted image with fat suppression. Note the marked distortion of the right globe and the native intracranial anatomy. The teratoma parasitized the brain’s blood supply, resulting in a large stroke and death
Glial–Neural Hamartomas
Glial–neural hamartomas occur rarely in the orbit and, therefore, often are difficult to diagnose. However, they should be considered as a possible diagnosis when an orbital mass is discovered at birth or shortly thereafter and lacks the characteristics of the more frequent pediatric orbital tumors, such as a capillary hemangioma, lymphangioma, dermoid, or teratoma. The glial–neural hamartomas may be well defined or infiltrative (Figs. 32.21 and 32.22) [59]. The presence of other congenital abnormalities (Fig. 32.22d) such as an abnormal globe, optic nerve, or extraocular muscles may be helpful in reaching the correct diagnosis by indicating maldevelopment as an etiology. However, if orbital structures appear normally formed and the glial–neural hamartoma is poorly defined (Fig. 32.21), then such a glial–neural malformation may be difficult to differentiate from fibromatosis or a rhabdomyosarcoma.




Fig. 32.21
A glial neural hamartoma that presented with a tonic pupil in a 4-month-old child. (a) Coronal T1-weighted MRI reveals an irregular mass (arrows) in the inferotemporal quadrant of the right orbit. (b) Coronal MRI following contrast infusion and fat suppression reveals enhancement of the mass (black arrows) that is isointense to the rectus muscles. Abnormal enhancement (white arrow) is also noted around the optic nerve–sheath complex and within intraconal fat


Fig. 32.22
Anophthalmos with neuroglial malformation . A degenerated optic vesicle resulting in anophthalmos with neuroglial malformation in the extraconal portion of the left orbit in an 8-month-old born without a left eye along with partial agenesis of the corpus callosum and an abnormal left cerebral hemisphere. (a) Coronal T1-weighted MRI shows a large superior extraconal mass (white arrows) displacing the orbital contents. The rectus muscles and optic nerve–sheath complex are markedly hypoplastic. (b) Sagittal T1-weighted MRI shows the mass (white arrows) occupying more than one-half of the orbit. The black arrow points to an abnormally enlarged subarachnoid space, posterior to the orbit and anterior to the temporal lobe. (c) Axial T1-weighted MRI through the superior orbits shows the extensive, slightly heterogeneous mass on the left. The intracranial left optic nerve (thin arrow) is atrophic compared to the right optic nerve (thick arrow). (d) Axial proton density MRI obtained through the inferior orbits shows the normal globe on the right and the most anterior–inferior portion of the mass on the left, which mimics a globe. Note that the signal intensity of the mass is similar to brain tissue and is higher than that of the normal globe. There is an irregular region of hypointensity (white arrow) just posterior to the mass, which is consistent with markedly atrophic abnormal left globe (secondary anophthalmos). The medial and lateral rectus muscles extend to this region. A very thin optic nerve–sheath complex (black arrow) can also be identified extending from the irregular tissue
Abnormalities of Extraocular Muscles
Strabismus (ocular misalignment) occurs frequently in children and involves various clinical patterns. Although the most common type of strabismus is congenital, other conditions may mimic it, and these may be related to neurologic disease, which should be promptly diagnosed and treated [60]. In this regard, imaging plays an important role for it can demonstrate cranial base and intracranial lesions responsible for cranial nerve palsies that result in paralytic strabismus. MRI can reveal and characterize cavernous sinus lesions, such as cranial nerve schwannoma (Fig. 32.23), inflammation (Fig. 32.24), or aneurysm (Fig. 32.25). In addition, brain parenchymal traumatic, infectious, inflammatory, demyelinating, ischemic, and neoplastic lesions responsible for cranial nerve palsies are well demonstrated by MRI even when they are very small and located within the brainstem [61].





Fig. 32.23
Oculomotor schwannoma . Right oculomotor schwannoma resulting in atrophy of extraocular muscles and right exotropia. (a) Axial T1-weighted MRI reveals deviation of the right globe laterally and a markedly thin right medial rectus muscle (arrow). (b) Contrast-enhanced coronal MRI of the cavernous sinuses reveals a small mass (arrows) of the right oculomotor nerve abutting the carotid siphon (seen as a flow void)

Fig. 32.24
Inflammation in the right cavernous sinus . (a) Axial T1-weighted MRI shows slight expansion of the right cavernous sinus (arrows) and marked narrowing of the intracavernous carotid artery. Also note the extensive opacification of the ethmoid and sphenoid sinuses due to paranasal sinus disease. (b) Axial T1-weighted MRI after gadolinium enhancement and fat suppression shows enhancement in the enlarged cavernous sinus and the narrowed carotid artery (arrow). Note the lack of definition of the anatomic structures at the apex of the right orbit in contrast to the normal left orbit


Fig. 32.25
Giant clotted aneurysm eroding the right orbital apex and displacing the optic nerve and chiasm. (a) Coronal T1-weighted MRI shows a multilobulated aneurysm with the medial lobule markedly displacing the chiasm (black arrow) and causing pressure erosion of the sphenoid bone (white arrow). (b) Parasagittal T1-weighted MRI shows the medial lobule of the aneurysm projecting into the sella turcica and elevating the chiasm (arrow). (c) On axial T2-weighted MRI, the large, round aneurysm with a hypointense wall, hyperintense inner rim, and central hypointensity erodes the apex of the right orbit. The right carotid artery is not identified; the left (arrows) is obvious
The excellent morphologic detail of the orbital structures provided by CT and MRI can also be helpful in the more complicated cases of ocular misalignment and fixation. Differential diagnosis can be provided between extraocular muscle absence (Fig. 32.26) [62], malfunction, and actual restriction due to fibrosis (Fig. 32.27) or fibrotic mass (Fig. 32.28). If there are complications after strabismus surgery (e.g., slipped muscle, excessive scarring) (Fig. 32.29), imaging can be utilized to assess the problem and to plan further management.





Fig. 32.26
Bilateral double elevator palsy . (a) Coronal T1-weighted MRI shows bilateral absence of the superior recti and atrophic superior oblique and medial rectus muscles. The location of the optic nerves (arrows) in the upper portions of the orbits is due to the resultant hypotropia. Compare this to a normal coronal of orbits shown in (b). (c) Parasagittal T1 MRI shows the globe in a hypotropic position, with absence of the superior rectus muscle. The arrow points to the optic nerve

Fig. 32.27
Congenital orbital fibrosis in an 11-month-old girl with enophthalmos since birth. (a) Axial T1-weighted MRI shows a thickened and irregular left medial rectus muscle (arrow). The left lateral rectus crosses the apex of the orbit to meet the short medial rectus muscle (open arrow). The lateral wall of the left orbit is shorter than that of the normal orbit on the right. (b) Coronal T1-weighted MRI reveals the fibrotic process causing obliteration of the margins and thickening of the extraocular muscles in the left orbit. There is abnormal widening of the superior extraconal space. The left orbit is enlarged and the left maxillary sinus is hypoplastic

Fig. 32.28
A 5-month-old child with right hypotropia due to fibrosing mass of the inferior rectus muscle . (a) Coronal T1-weighted MRI shows marked asymmetry in the position of the lenses. The right eye is fixed in downward gaze position. (b) Sagittal T1-weighted MRI reveals a soft tissue mass (arrows) along the course of the inferior rectus

Fig. 32.29
Severe ocular malalignment with marked thickening of the left medial rectus muscle. The patient had multiple previous surgical procedures for strabismus. (a) Coronal T1-weighted MRI shows marked thickening of the right medial rectus muscle (arrow). The optic nerve/sheath complex (open arrow) is elevated (compare to the right side). (b) Coronal T1-weighted image shows the left globe turned inferomedially (note the grossly abnormal position of the lens). The globe is also surrounded by irregular fibrotic tissue. (c) Axial T2-weighted MRI shows the abnormal position of the globe with the lens (arrows) directed medially
Traumatic Orbital Lesions
The type and frequency of orbital traumatic lesions that occur in children depend on the age of the child. Young children (<4 years) manifest atypical orbital fractures from blunt trauma for two reasons. First, the cranial-to-maxillofacial ratio in infants is very high (8:1) and progressively decreases to 2.5:1 in adulthood [63–65]. Younger children also lack pneumatized frontal sinuses, which act to distribute forces applied to the forehead. Young children therefore are especially prone to orbital roof fractures, which may result in intracranial and orbital subperiosteal hemorrhages, as well as cerebrospinal fluid leakage into the orbit (Fig. 32.30) [63, 64, 66–68]. Because of the flexibility of the young cranial bones and the background pulsatility of the brain, a “growing fracture” of the orbital roof may result. Older children and teenagers have a spectrum of traumatic lesions similar to that of adults, including blowout, tripod, and midface fractures [68]. One important exception to this adult pattern is the propensity of older children and teenagers to sustain greenstick fractures of the orbital floor and medial wall (Fig. 32.31), also known as the “white-eyed blowout fractures” [69–71]. Imaging in such cases may appear deceptively normal, since the trapdoor nature of the fracture snaps back into normal position immediately after the injury [70, 71]. However, patients with WEBOF often present with severe restrictive external ophthalmoplegia and vasovagal symptoms misdiagnosed as concussion because of tight and ischemic entrapment of the extraocular muscle (EOM) adjacent to the fracture. WEBOF represent an ophthalmic emergency; delay in release of the entrapped muscle may result in permanent ischemic injury and strabismus. WEBOFs are best diagnosed on coronal views, with careful attention to the course and contour of the adjacent EOM (Fig. 32.32). Sagittal images can also be helpful in delineation of the inferior and superior rectus muscles.







Fig. 32.30
Orbital roof fracture with resultant subperiosteal hematoma . (a) Clinical photograph of a 2-year-old child following blunt trauma. (b) CT, coronal soft tissue window shows a small subperiosteal hematoma (arrow). (c) CT, parasagittal bone window reveals a small fracture (arrow) in the orbital roof. (d) Graphic representation of specific fracture incidence as a percentage of all pediatric orbital and midfacial fractures across multiple age groups (Data compiled from Ref. [64])


Fig. 32.31
Medial WEBOF in a young teenager. (a) Note the severe horizontal gaze restriction of the right eye. (b) On coronal bone window CT, a narrow fracture in the right lamina papyracea is noted (arrow). (c) Coronal soft tissue window CT shows a marked distortion of the right medial rectus muscle (arrow) compared to the left, consistent with entrapment of the muscle within the confines of the fracture

Fig. 32.32
WEBOF and the “missing muscle sign.” A teenager was struck in the left orbit with a baseball. He immediately complained of nausea, was diagnosed with a concussion, and underwent head CT. After subsequent transfer, an orbital CT was performed. (a, b) Coronal soft tissue windows on CT of the orbits. Note that the left inferior rectus appears to be missing but has actually herniated into the maxillary sinus (arrows) along with some surrounding radiolucent fat. A narrow fracture is present. (c) On more posterior cuts, the inferior rectus (between the arrows) is incarcerated within the trapdoor fracture, with resultant ischemia
Penetrating orbital injury is more common in children than adults and is particularly frequent in younger children, who often tend to fall while playing or running with sharp objects or toys. Evaluation of very young children who have sustained penetrating orbital injury may be difficult due to lack of cooperation as well as lack of exact history and information as to type of trauma and type of penetrating object. The presence of a foreign body may not be externally obvious. Furthermore, a detailed history is often difficult to obtain. There may be both superficial and deep components of a foreign body, and organic (wooden) FBs may be present. Therefore, it is important to evaluate the entire orbit as well as the adjacent structures, the paranasal sinuses, and brain.
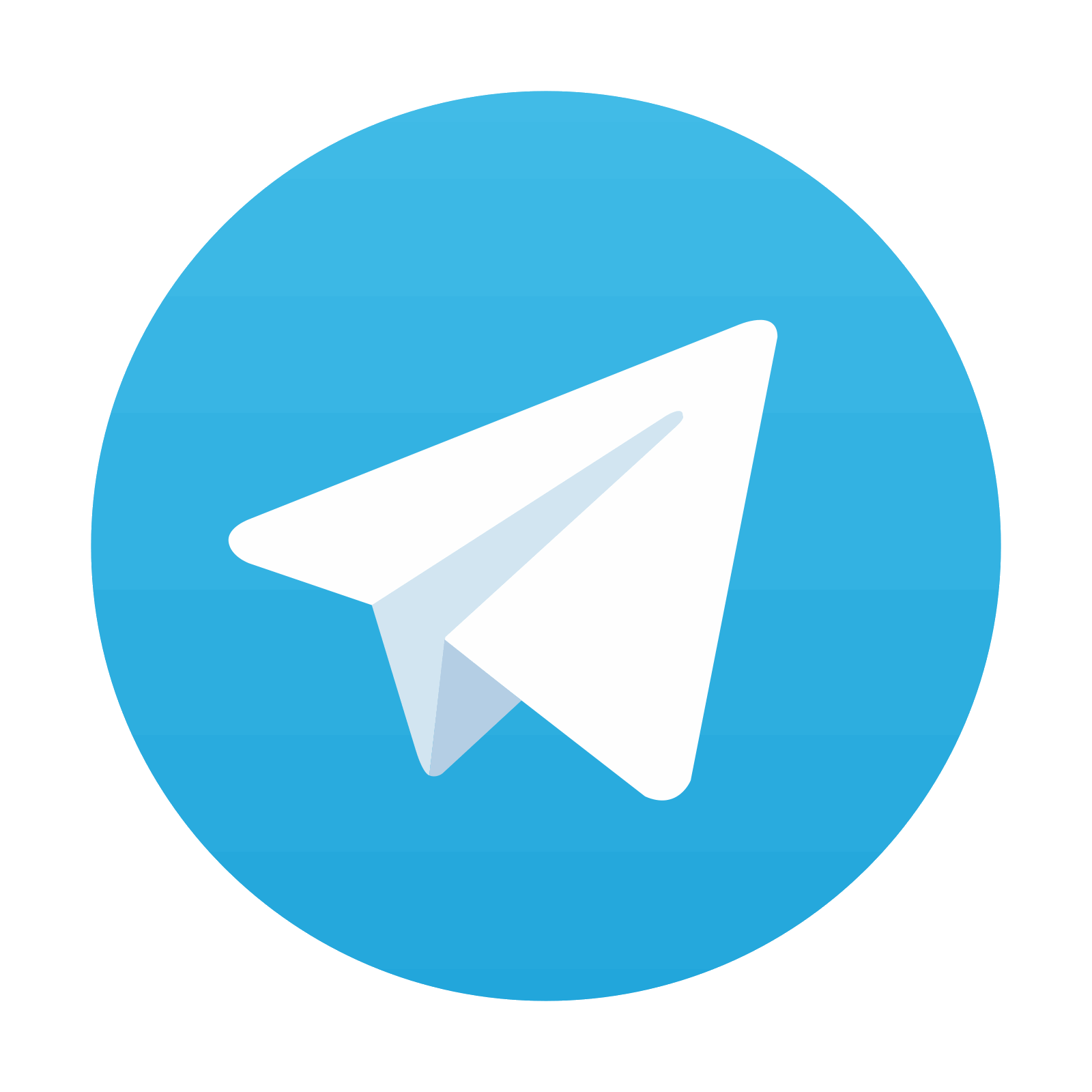
Stay updated, free articles. Join our Telegram channel
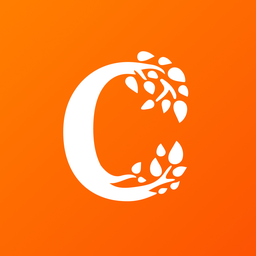
Full access? Get Clinical Tree
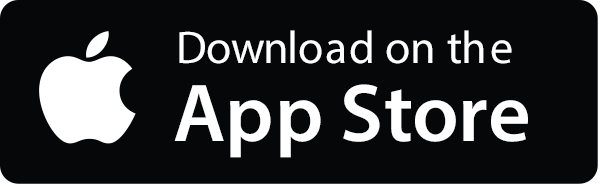
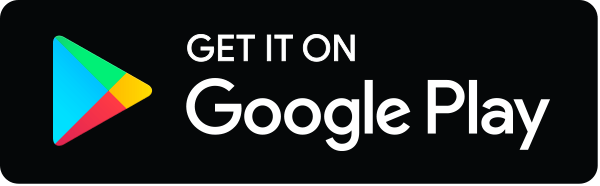