, Maneli Mozaffarieh1 and Hans Bebie2
(1)
Department of Ophthalmology, University of Basel, Basel, Switzerland
(2)
Institute for Theoretical Physics, University of Bern, Bern, Switzerland
Abstract
An ophthalmologist has the advantage that the most frequent pathological findings can be seen directly or imaged by means of light. Nevertheless, ophthalmologists sometimes also need to use additional imaging procedures, though less frequently than a neurologist, for example. Here, we will discuss conventional radiography, digital radiography, computed tomography (CT), and magnetic resonance imaging (MRI).
An ophthalmologist has the advantage that the most frequent pathological findings can be seen directly or imaged by means of light. Nevertheless, ophthalmologists sometimes also need to use additional imaging procedures, though less frequently than a neurologist, for example. Here, we will discuss conventional radiography, digital radiography, computed tomography (CT), and magnetic resonance imaging (MRI).
Radiography and CT are based on X-rays. These are “hard” electromagnetic radiation with photon energies far beyond those of visible light. They pass through the body and, while doing so, are more strongly attenuated by bony structures than by other tissues. MRI is based on the interactions between “soft” electromagnetic pulses and atomic nuclei, primarily hydrogen nuclei.
6.1 Analog Radiography
The discovery of X-rays in 1895 by Röntgen1 was one of the greatest advances in medical technology (Fig. 6.1). For the first time, it was possible to image bones in the bodies in vivo. In very short order, his discovery received a great deal of attention worldwide. The image was recorded by a photosensitive layer (emulsions on the glass plates used in black-and-white photography at the time). The function of the photographic plate – and later film – as the detector and for long-term documentation continues today.
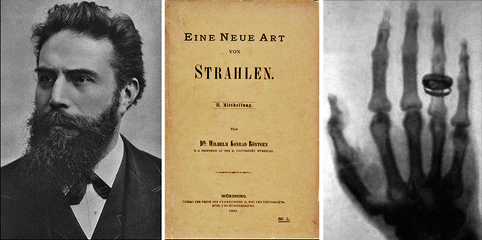
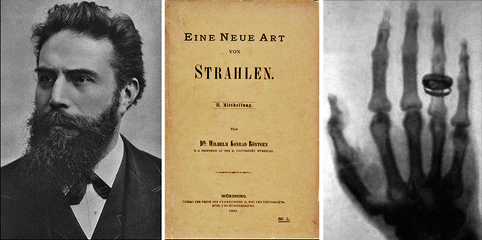
Fig. 6.1
The title of Röntgen’s first publication, A New Kind of Radiation, indicates that the nature of the X-rays was unexplained in the beginning. Right: One of the first X-ray photos
Expressed in today’s language, Röntgen experimented with electrons that were accelerated in a vacuum tube by a high electrical voltage to make them collide with a positively charged electrode (anode), where they engender electromagnetic radiation of very short wavelengths (photons with high energies). This principle has remained unchanged right up to the present time. Röntgen’s electrons originated from the gas remaining in the tubes and the negative electrode (cathode) was cold. Considerably higher and adjustable power resulted later with hot cathodes from which electrons could easily emerge (Fig. 6.2).
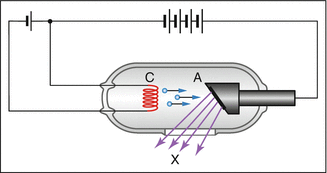
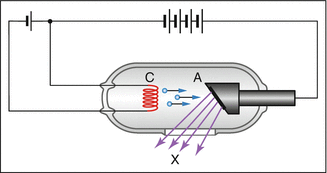
Fig. 6.2
Principle of the X-ray tube. The cathode (C) glows. A high voltage between the anode (A) and the cathode accelerates electrons. Their collisions with the anode give rise to high-energy photons (X-rays)
Typical operating voltages are in the range from 30 to 120 kV. Ideally, an electron transfers its whole energy to a single photon. The maximum energy of the photons created with a voltage of 30 kV, thus, amounts to 30,000 eV, approximately 10,000 times higher than the energy of a blue light photon. Correspondingly, the wavelength is shorter than that of blue light by the same factor (Table 6.1). In the language of electrodynamics, the generation of photons is based on the acceleration of electrons. Here, it is the deceleration (sudden stopping) that is involved; i.e., a negative acceleration. Part of the kinetic energy of the electrons is transformed into electromagnetic radiation (photons) and part contributes to heating of the anode.
Table 6.1
X-rays. The relationship between the electrical operating voltage, wavelength, and maximum energy of the photons. 1 kV = 1000 V (for comparison with blue light photons: energy = 3 eV, wavelength = 400 nm)
Operating voltage (kV) | Wavelength (nm) | Maximum photon energy (eV) |
---|---|---|
30 | 0.04 | 30,000 |
120 | 0.01 | 120,000 |
Not all photons exhibit the maximum possible energy. Figure 6.3 shows a typical spectrum. The choice of voltage between anode and cathode influences the mean energy of the Röntgen quanta: the higher their energies are, the better they penetrate through sections of the body; i.e., the attenuation is less. X-rays damage tissue. Studies have shown that the additional risk for cancer due to X-ray examinations differs in industrial nations but may be on the order of roughly 1 %.
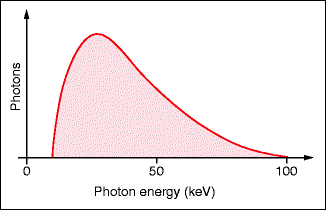
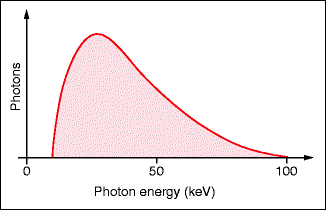
Fig. 6.3
Typical distribution of photon energies. The maximum is determined by the operating voltage of the X-ray tube (Table 6.1). Operating voltage: 100 kV
6.2 Digital Radiography
Digital radiography is slowly replacing the analog form. The difference does not lie in the way that X-rays are generated or in the interactions between the rays and the tissues but in how the X-ray images are recorded. Instead of photosensitive film, reusable flat-panel detectors are employed.
Among the various technologies, we will discuss the elements of a system that functions with an X-ray storage panel (Fig. 6.4). The X-rays are generated in the traditional way. They pass through the body that is being examined and the whole image cross-section simultaneously falls onto the X-ray flat-panel detector that has replaced photosensitive film. Rather than a photosensitive emulsion, the panel carries an approximately 0.1 mm thick photostimulatable phosphor layer that is able to receive an X-ray intensity image and store it for a period of time. This amazing process begins with the layer’s electrons being pushed up to a higher energy level by the X-ray photons. If more X-rays arrive in parts of the image, a greater number of electrons undergo this transition. Instead of returning spontaneously to the previous level, these electrons are held captive in an excited state by the phosphor layer. This state can last for hours. It is only when the panel is illuminated with light that the electrons return to their original state, thereby immediately emitting fluorescent light. This reading process occurs in a separate reading unit where a laser beam scans the X-ray storage sheet line by line. A light-sensitive photodetector registers the temporal sequence of the fluorescent light. This signal is digitized and the information is stored in a computer.
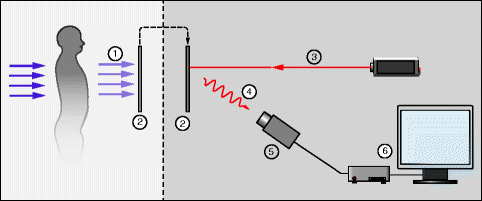
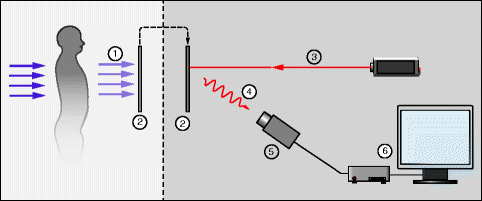
Fig. 6.4
Principle of digital radiography. The X-ray picture (1) is acquired and stored on a plate (2) containing crystals of a photostimulatable phosphor (e.g., BaFBr). Later, the image can be read with a scanning laser beam (3). Pulses of visible photons are produced, point by point, depending on the X-rays that were received (4). They are then detected by a photomultiplier or photodiode (5). A computer (6) records the image in the form of the point-by-point number of these photons. The resolution is on the order of 2,000 × 2,000 pixels with a dynamic range of 10 bits
The X-ray storage sheet is reusable. The storage of large X-ray films is no longer necessary. The digitally stored data permit post-processing of the image, e.g., contrast improvement and, thus, a certain independence from insufficient exposure. Compared to film radiography, the radiation dosage can be reduced to some extent while retaining similar image quality. The disadvantages include the investment required for the reading unit and the dependence on computer systems.
In Fig. 6.5, a dacryocystography is shown as an example of a classical radiograph, enhanced with a contrast dye.
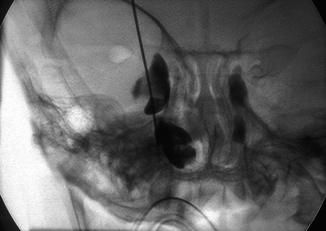
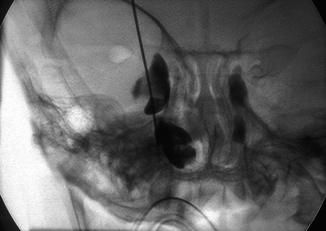
Fig. 6.5
Distal occlusion of the lacrimal duct visualized by dacryocystography. (Courtesy of L. Remonda, Kantonsspital Aarau, Switzerland)
6.3 Computed Tomography (CT)
The invention of computed tomography in the 1970s was another great breakthrough in diagnostics. Instead of silhouettes, sectional images through body parts are generated in which the forms and positions of the organs are displayed.
The basis of this method is also X-rays. The information for a section image is obtained by a thin sheet of X-rays that initially pass through the body in a certain direction. For this direction of irradiation, a series of detectors records a one-dimensional image of the attenuation of the radiation as a function of the transverse coordinate. These recordings are then repeated in the same sectional plane for a large number of directions (Fig. 6.6). From the totality of information acquired, a computer reconstructs the distribution of the attenuation coefficients within the irradiated section, thereby producing an image of the tissue distribution in that section.
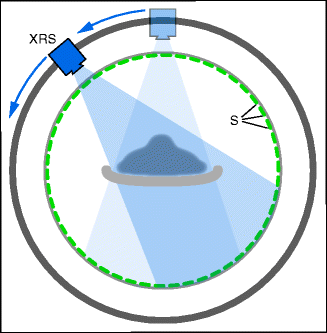
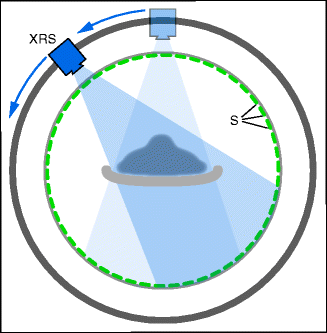
Fig. 6.6
Recording method in computed tomography. A sheet of X-rays passes through the body from a given direction. One-dimensional recording as a function of the transverse coordinate. Repetitions from many directions result in a section image. XRS: X-ray source. S: Sensors
Normally, several adjacent sectional images are procured. With spiral-CT, the recording system rotates with continuous radiation without interruption during the entire examination of the patient. At the same time, the patient table is also moved along continuously. This type of scan produces a spiral-shaped collection of transmission data with an unbroken recording of the region being examined. The data carry three-dimensional information that can be displayed as a calculated section image, where any position of the section plane can be chosen within the examined region. A clinical example of a CT of the orbita in a patient with head injury is shown in Fig. 6.7. The CT allows particularly clear visualization of the bones.
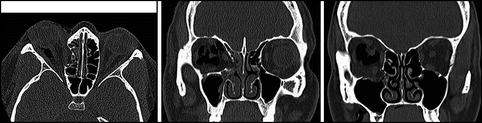
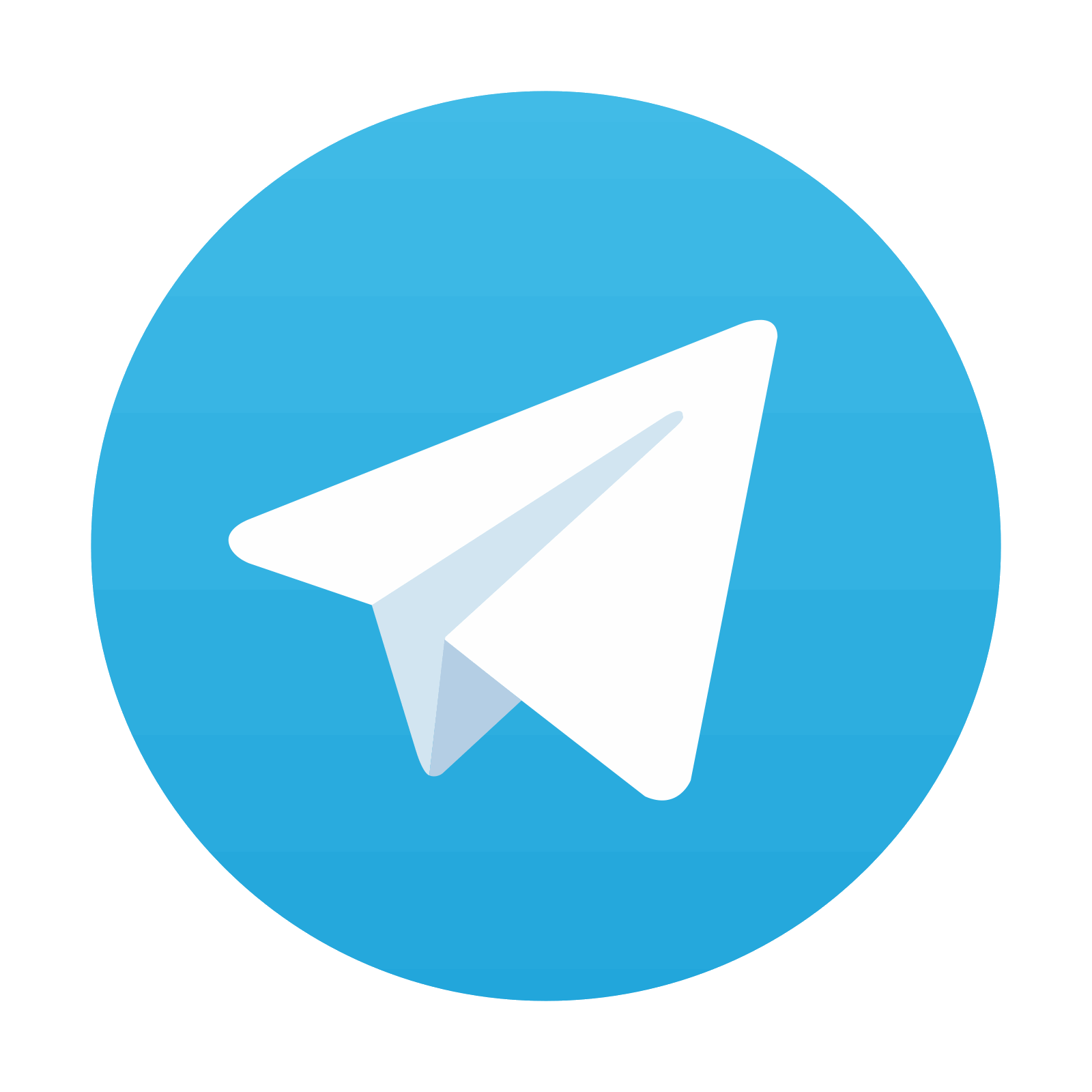
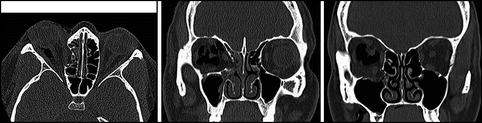
Fig. 6.7
Orbita CT revealing impression of the lamina papyracea, impression of the orbita floor, and an intraorbital hematoma. Left: axial section. Middle and right: coronal sections (Courtesy of L. Remonda, Kantonsspital Aarau, Switzerland)
< div class='tao-gold-member'>
Only gold members can continue reading. Log In or Register a > to continue
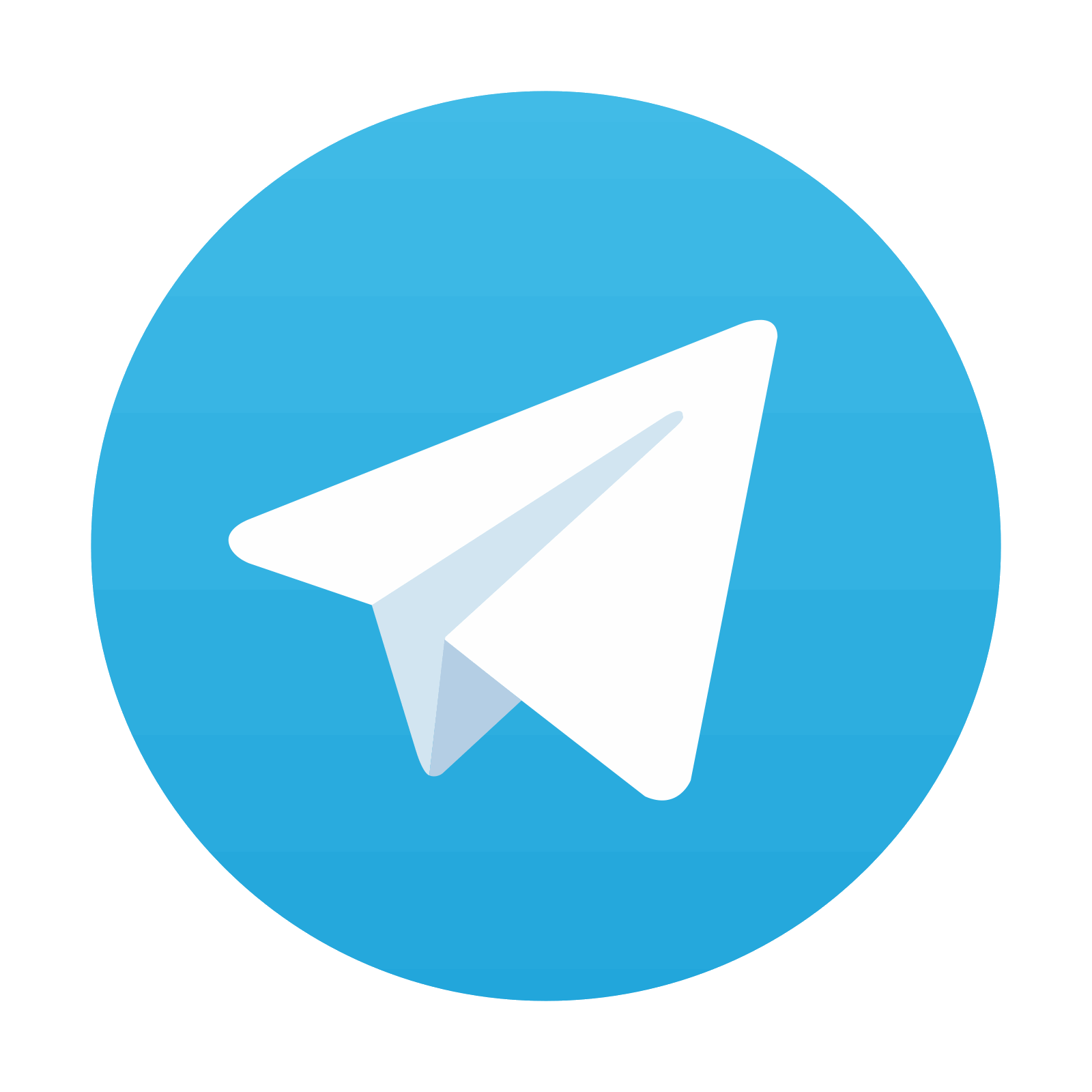
Stay updated, free articles. Join our Telegram channel
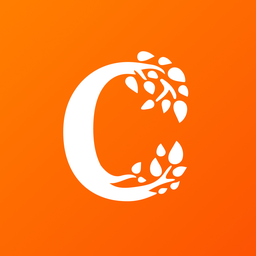
Full access? Get Clinical Tree
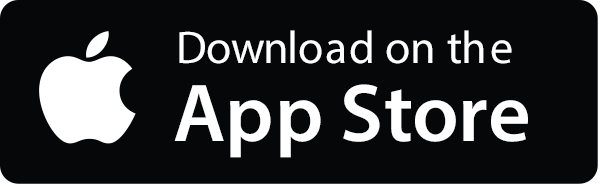
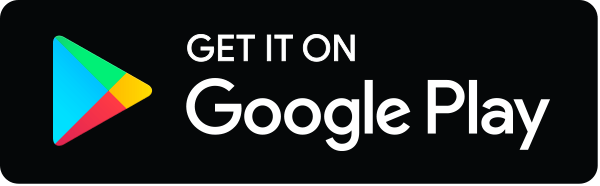
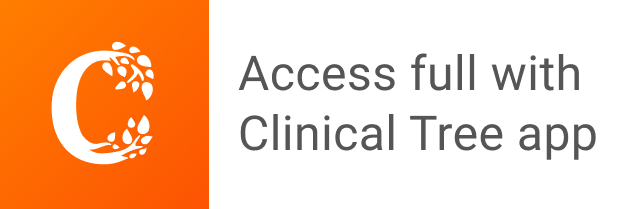