, Maneli Mozaffarieh1 and Hans Bebie2
(1)
Department of Ophthalmology, University of Basel, Basel, Switzerland
(2)
Institute for Theoretical Physics, University of Bern, Bern, Switzerland
Abstract
We now turn to the role of light as the most important “instrumentarium” for ophthalmological examinations. In Sect. 4.1, we discuss methods that are based on geometrical optics, such as, for example, ophthalmoscopes, contact lenses, and slit lamps. The appearance and functions of today’s instruments should be quite familiar. We primarily present the optical principles, but we also intersperse a few historical illustrations. Optical coherence tomography (OCT) utilizes the wave nature of light and, for this reason, we shall treat its elements in a separate section (Sect. 4.2). We shall also dedicate a section (Sect. 4.3) to discussing optical Doppler effects, which allows movement to be observed.
We now turn to the role of light as the most important “instrumentarium” for ophthalmological examinations. In Sect. 4.1, we discuss methods that are based on geometrical optics, such as, for example, ophthalmoscopes, contact lenses, and slit lamps. The appearance and functions of today’s instruments should be quite familiar. We primarily present the optical principles, but we also intersperse a few historical illustrations. Optical coherence tomography (OCT) utilizes the wave nature of light and, for this reason, we shall treat its elements in a separate section (Sect. 4.2). We shall also dedicate a section (Sect. 4.3) to discussing optical Doppler effects, which allows movement to be observed.
4.1 Methods on the Basis of Classical Optics
4.1.1 The Ophthalmoscope (Direct Ophthalmoscopy)
From a purely optical point of view, two emmetropic people could quite possibly see the other’s retina by looking through each other’s pupils (Fig. 4.1). The reason that this does not work in real life is the lack of illumination. The light that exits the eye of the person sitting opposite is so weak that the pupil appears black. The great contribution of Helmholtz1 was the fact that he was able to illuminate the interior of a patient’s eye in a way that the observer could still look through the patient’s pupil. Semitransparent mirrors did not exist back then. To achieve a similar effect, he used a stack of several plates of glass. The reflection of these glass plates made it possible to guide light into the patient’s eye while, at the same time, the physician could look through them. He saw an upright and non-reversed image of the retina.
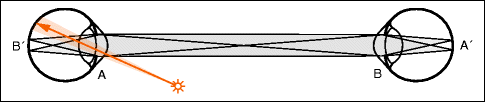
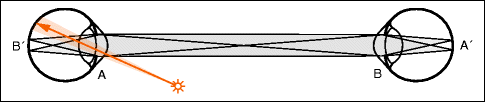
Fig. 4.1
Eyes seeing each other. A′ and B′ are the images of the pupils A and B, respectively (mutual focusing on the observed pupils and ideal imaging are assumed). The light that pupil B receives from pupil A originates exactly from the image B’. Without a light source between the two eyes, the images A′ and B′ remain unilluminated (disregarding possible stray light from other parts of the eyes). Arrow: a source of light that does not lie between the two pupils is unable to brighten the images
The fact that the pupil appears black has several causes. When light comes to us from all directions, the light intensity at the level of the retina, with a pupil diameter of 4 mm, is roughly 70 times less than that at the level of the iris. In addition, strong absorption also occurs: the fundus absorbs roughly 90 % of red light and roughly 99 % of blue light. Therefore, inside an eye, a darkness is present that is very similar to that inside a room with a small window and brown walls. In light of observations of the fundus – visually or photographically – the blackness of the pupils can also be explained as follows: depending on a photon’s color, when it enters the eye, it has a probability of just 10−4 to 10−3 of coming out again.
In 1851, when Helmholtz invented the ophthalmoscope, he knew about its practical importance (Figs. 4.2 and 4.5). Ten years later, the ophthalmoscope and the observations that had become possible with it were already the subjects of several dozen publications. With the help of this ophthalmoscope, von Graefe2 was the first to describe optic nerve head excavations in glaucoma (Fig. 4.3).
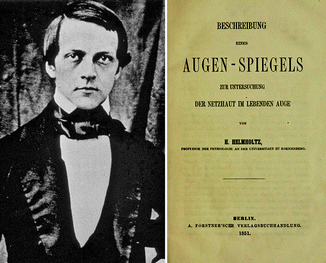
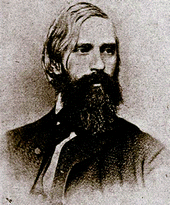
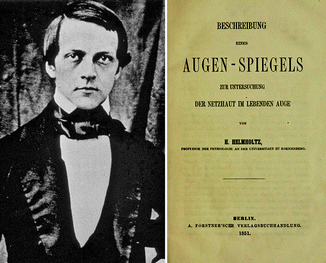
Fig. 4.2
Helmholtz and his 46-page monograph “Beschreibung eines Augen-Spiegels zur Untersuchung der Netzhaut im lebenden Auge” (“Description of an Eye Mirror for Examining the Retina in Living Eyes”) (1851). It is devoted to optics, handling, and the first physiologic observations. It is available online: www.archive.org
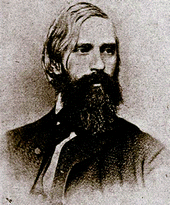
Fig. 4.3
Albrecht von Graefe
The light path of direct ophthalmoscopy is shown in Fig. 4.4. The examiner views the patient’s fundus through the patient’s optical system, which has the effect of a positive lens and leads to a magnification of the funduscopic image by a factor of approximately 14. To enlarge his viewing angle, the examiner has to come very close to the observed object. If we are used to viewing the retina by means of direct ophthalmoscopy, we are surprised at how small the actual retina is in an anatomic preparation when viewed by the naked eye from a distance of 25 cm. If either the patient or the examiner is ametropic, this is corrected by the addition of a small lens that is brought into the observation path (these lenses are built into the so-called Rekoss disk) (Fig. 4.5).
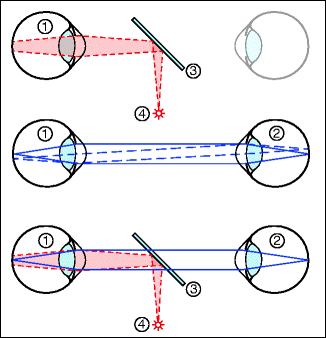
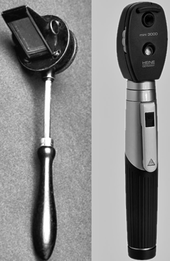
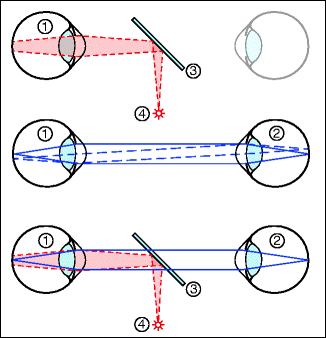
Fig. 4.4
Principle of direct ophthalmoscopy. Top: the illumination (4) is introduced via a semi-transparent mirror (3). With a point light source from a distance near the eye, the field of view can be illuminated. The cornea and lens give rise to reflections. Middle: observation pathways. The patient’s retina (1) is imaged one to one on the retina of the observer (2). A correction lens, used to correct for possible refraction deficits, is not shown (in the case of two emmetropic eyes, no optical corrections are necessary). The field of view is limited by the diameter of the pupil; it gets wider the closer the two eyes are positioned in relation to one another. Bottom: both pathways combined
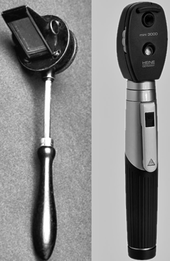
Fig. 4.5
Direct ophthalmoscopes. Left: construction according to Helmholtz. Right: modern version
White light is normally used for examinations of the eye. This enables the examiner to recognize not only shape alterations but also changes in color. For specific questions, certain parts of the color spectrum are preferable. For example: if we would like to see the retinal nerve fiber layer, blue light is superior for two reasons. First, the nerve fiber layer backscatters very little light. Of the amount of light reflected, blue is reflected more than any other color. In addition, most absorption of blue light takes place through the choroid, so minimal light is scattered back from there and it does not outshine the image of the nerve fiber layer. Therefore, corresponding filters are even more important in fundus cameras. For example, a blue interference filter (SE-40) of 495 nm wavelength is used for photographing the nerve fiber layer (Fig. 4.6).
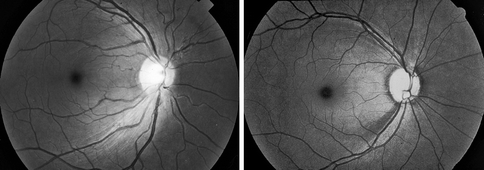
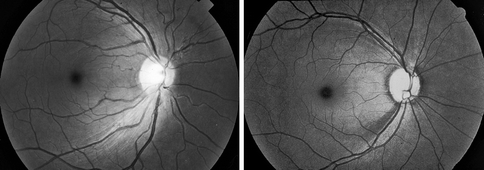
Fig. 4.6
Fundusphotography made through a blue-interference filter. Left: normal eye; right: nerve fiber bundle defect in a glaucomatous eye
Red-free green light is almost completely absorbed by hemoglobin and, for this reason, it gives better contrast of the blood columns in the blood vessels against the surrounding tissues. Hemorrhages are also easier to see. The dyes of the macula lutea (lutein and zeaxanthin) also strongly absorb this type of light and make it easier for the examiner to find the macula (Fig. 4.7).
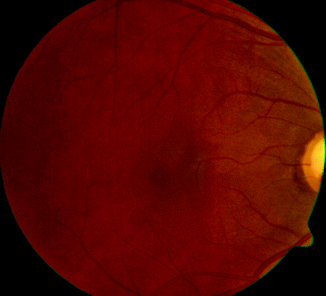
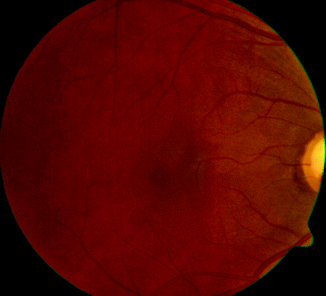
Fig. 4.7
The macula lutea appears slightly yellow due to the pigments lutein and zeaxanthin, which absorb blue light in particular
The disadvantages of the Helmholtz’s ophthalmoscope were reflections, which originated mainly from the cornea. Here, Gullstrand3 brought about a decisive improvement (Fig. 4.8). The illumination path was separated from the observation path so that, on the one hand, the reflections were eliminated, while, on the other hand, the view through an enlarged pupil was still possible (Fig. 4.9).
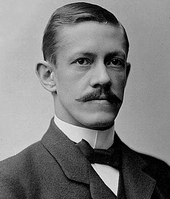
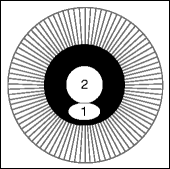
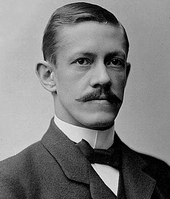
Fig. 4.8
Allvar Gullstrand
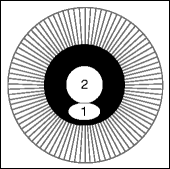
Fig. 4.9
Principle of separating the illumination pathway (1) from that of the observation (2) in the pupil and lens of the patient. Central ophthalmoscopy (observation through the center of the pupil) results in the best image resolution but requires a wide pupil
4.1.2 Indirect Ophthalmoscopy
Indirect ophthalmoscopy allows monocular and binocular (stereoscopic) observation of the patient’s retina with a variety of magnifications and fields of view. For indirect ophthalmoscopy, illumination of the retina is also necessary, but this will not be discussed here. A collecting lens in front of the patient’s eye creates a real, mirrored, and inverted image of the ocular background that is then observed by the physician (Figs. 4.10 and 4.11). From a distance of 25 cm and with a lens of 70 mm focal length, the ophthalmologist observes an image of the retina that is approximately three times smaller than the image in direct ophthalmoscopy. In return, indirect ophthalmoscopy offers a substantially larger field of view. In terms of enlargement and field of view, direct and indirect ophthalmoscopes complement each other.
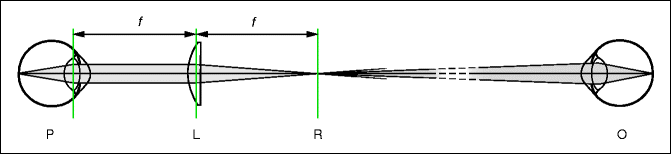
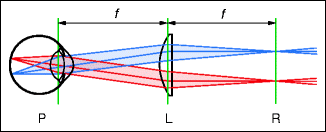
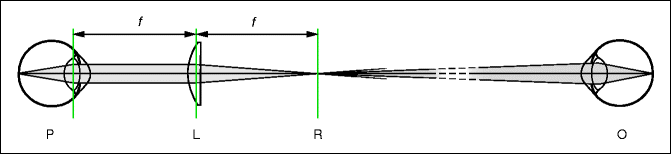
Fig. 4.10
Central beam paths of monocular indirect ophthalmoscopy. P patient’s eye, L strong convex lens, R real inverted and mirrored image of the patient’s retina, O observer. The distance between L and R is given by the focal length of L
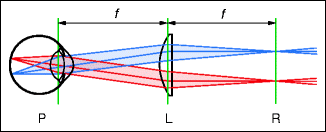
Fig. 4.11
Indirect ophthalmoscopy. The field of view is optimal when the distance of the convex lens (L) from the nodal point of the eye is the same as the focal length of L. The nodal point of the eye lies roughly in the center of the crystalline lens. In this arrangement, the field of view is determined only by the edge of L. A stronger lens yields a smaller image and a wider field of view
In 1947, Schepens4 developed the binocular indirect ophthalmoscope, which also contained the illumination. Figure 4.12 shows that the light beam in the observer’s direction is narrow. It becomes narrower as the patient’s pupil gets smaller and as the focal length of the lens gets larger. The two eyes of the observer are able to access the light beam simultaneously only with the aid of prisms. Since the stereo angle α is small, the stereoscopic effect is not very large (Fig. 4.13).
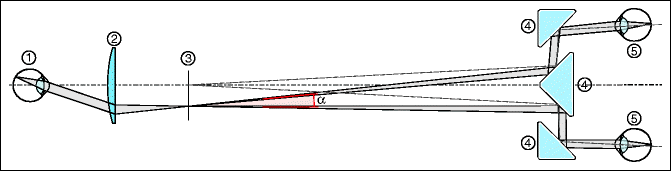
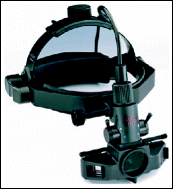
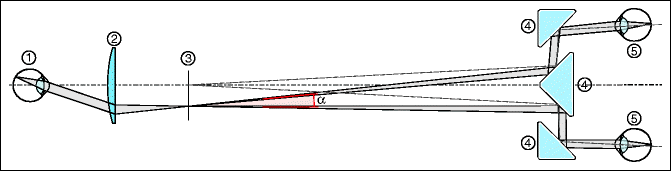
Fig. 4.12
Binocular (stereoscopic) indirect ophthalmoscopy. Just as in monocular indirect ophthalmoscopy, the lens (2) produces a real image (3) that is observed by the physician. The narrowing of the beam pathways with the aid of prisms or mirrors (4) is necessary so that the beam of light that emerges from the real intermediate image (3) reaches both eyes of the physician. Thus, the stereoscopic impression, although diminished, is still present and helpful for the surgeon. In this figure, the distance of the examiner from the intermediate image is shown too short; in reality, it amounts to about 50 cm. The magnification from retina to retina is given by the ratio of the focal length of the lens (2) to the distance of the ophthalmologist from the intermediate image. In this figure, it is roughly 0.15, approximately 6 times smaller than in direct ophthalmoscopy (1:1) – this is the price for the larger field of view. α stereo angle
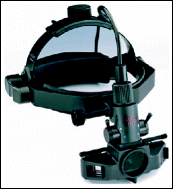
Fig. 4.13
Indirect ophthalmoscopy according to Schepens. The illumination is built-in
4.1.3 The Slit Lamp
The slit lamp was developed by Gullstrand5 in 1911. The improvements made by Goldmann6 contributed decisively to its popularization as a standard instrument. In all models, the microscope (observation unit) and the illumination unit are combined. The microscope can be positioned in all three dimensions, independent of the direction (and tilting angle) of the illumination (Fig. 4.14). Two modes of observation are possible: focal, i.e., direct observation of an illuminated structure, or confocal, whereby the scattered light that arises in the illuminated slit is observed obliquely from the side (see the figures in Sect. 2.7).
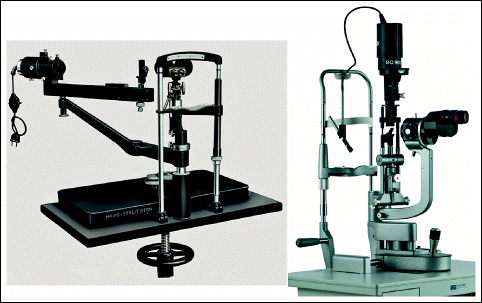
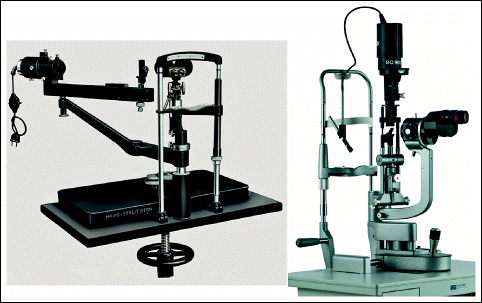
Fig. 4.14
Left: Goldmann slit lamp (1933). One recognizes the stereomicroscope and the projector for the slit light. Right: modern model. (Courtesy of Haag-Streit AG, Switzerland)
The slit lamp biomicroscope enables stereoscopic observation with variable magnification (typically 6× to 40×). The optics fulfill the requirements of an upright image while leaving enough space for manipulations in front of the examined eye. A large depth of focus is desirable. The optics correspond to the principle of the telescopic magnifier. The optics can be completely separated for the two eyes, but instruments with common object-side magnifiers are also possible (Fig. 4.15). In both cases, the two eyes of the observer view the object under a stereo angle. This produces the stereoscopic effect. In Sect. 4.1.5, we shall explore the application of the biomicroscope for observing the fundus.
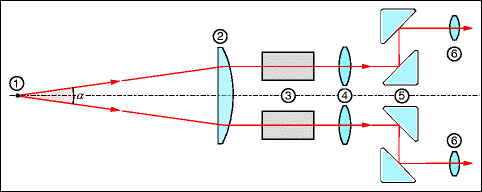
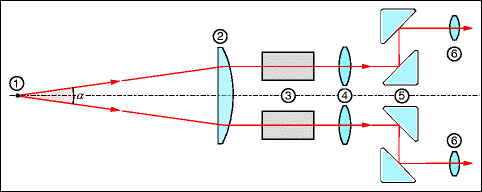
Fig. 4.15
Ray pathways of a slit lamp microscope according to the principle of the telescopic magnifier. In the construction sketched here, the beam paths for the two eyes of the observer separate only behind the magnifier (2). The magnifier (2) images the object (1) at infinity. Observation is performed with prisms (5) and with the telescope, consisting of an objective (4) and an ocular lens (6). The telescopic system (3) is used to alter the magnification. The stereo angle α equals 10° to 14° and corresponds to a stereoscopic view such as from a distance of 25 cm
The illumination unit produces a very bright slit light with adjustable elevation, width, position, and angular orientation. A very homogeneous illumination is desired; this is a task that is accomplished very well by the beam path, according to Köhler, known from the microscope (Fig. 4.16). The color of the slit light can be selected with filters. A large blue portion is able to produce sufficient stray light in the transparent media and also bring about fluorescence effects. The use of red-free light is further addressed in Sect. 4.1.
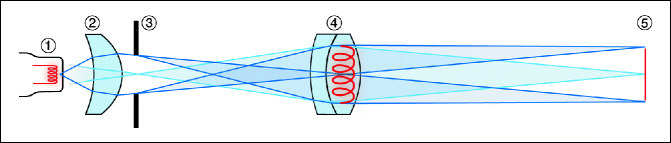
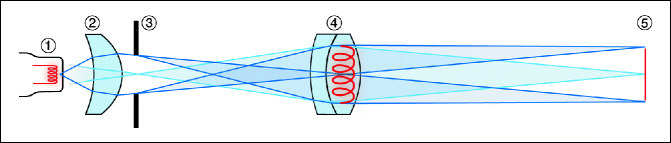
Fig. 4.16
Slit illumination according to Köhler. The collimator (2) images the filament (1) of an incandescent lamp through a slit aperture (3) into the objective lens (4). The objective images the slit, the dimensions and angular orientation of which are variable, into the slit light (5). The image of the filament does not have to lie precisely in the objective lens − what is important is that it is not imaged sharply by the objective (4) into the slit image (5)
At this point, we are not going to discuss any of the highly developed accessories for slit lamps, such as tonometers, pachymeters, cameras, or the optics for introducing laser radiation. Gonioscopy and funduscopy are mentioned in separate chapters, and Goldmann’s tonometry was discussed in detail in Sect. 2.9.
4.1.4 Contact Lenses
Contact lenses of different constructions permit the viewing of various regions and structures of the eye (Fig. 4.17). A contact lens essentially neutralizes the refractive power of the cornea. In Sect. 2.5, we showed how viewing into the anterior chamber angle is possible with a suitable contact lens (gonioscopy7). The Goldmann 3-mirror lens permits viewing angles on the whole inside of the eye (Fig. 4.18). The mirrors are tilted at angles of 59°, 66°, and 73°. The outermost periphery can be viewed only when the sclera is indented with the so-called indentation contact lens, which contains a scleral depressor. Contact lenses made of acrylic material have a somewhat higher index of refraction than the eye media (n = 1.49). The contact lens neutralizes approximately the refractive power of both the cornea and the crystalline lens.
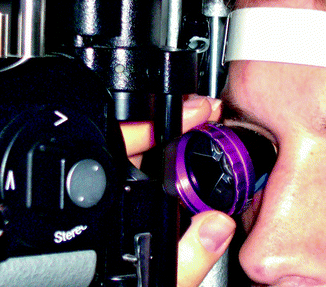
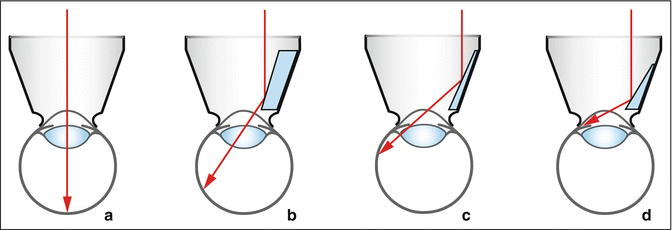
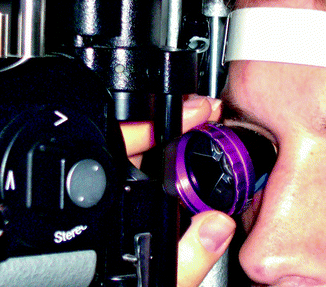
Fig. 4.17
Patient’s eye is examined using the slit lamp microscope through a contact lens
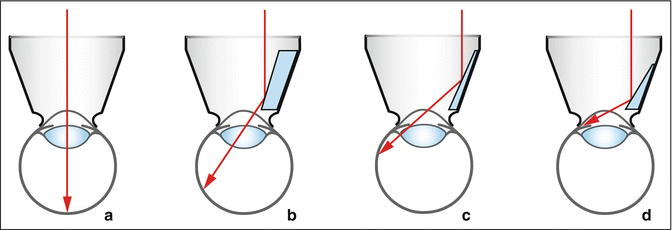
Fig. 4.18
Direct view and views through variously tilted mirror contact lenses. (a) Direct fundus examination. (b) With the 73° tilted mirror. (c) Mirror with a tilt of 66°; observation of the peripheral fundus. (d) Mirror with a tilt of 59°; observation of the very peripheral fundus and of the chamber angle (gonioscopy)
4.1.5 Funduscopy with the Slit Lamp
It is often said that the slit lamp was created mainly for examination of the anterior segment. This is true since strictly optical observations of the anterior segment are easy to perform and do not create a problem. Due to the strong refractive power of the cornea and lens, direct observation of the retina is not possible. Various methods have been developed to view the retina directly with the slit lamp. The direct view through a contact lens (e.g., Goldmann’s 3-mirror lens) allows inspection of the fundus with the slit lamp microscope.
The retina can also be examined by the slit lamp when using stronger lenses with a refractive power of, for instance, 78 or 90 D that are not in direct contact with the patient’s cornea (Fig. 4.19). Just as with indirect ophthalmoscopy, the lens then produces a real, mirrored, and inverted image (see Figs. 4.10 and 4.11). In contrast to indirect ophthalmoscopy, one views this image with a microscope. It is true that the 78 D lens minifies the retinal image compared to the original by a factor of 0.75 (60/78). However, the biomicroscope’s 10-fold magnification results in a net 7.5-fold magnification.
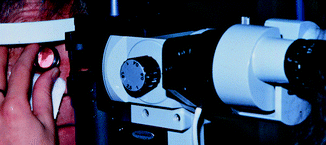
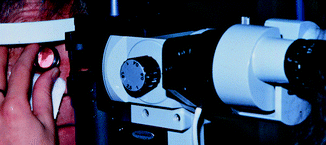
Fig. 4.19
Funduscopy with a 90 D magnifier
Another possibility is the insertion of a minus lens (lens according to Hruby8). With a refractive power of about −60 D, the eye’s refractive power is neutralized. The physician then sees an upright image of the retina.
The stereo angle of slit lamps amounts to about 12°–15°. There are models that permit a reduction of the angle to roughly 5°. This can make observations of the fundus easier – for example in a patient with a small pupil – and it enlarges the binocular field of view. However, the stereo effect is weakened.
4.1.6 The Operating Microscope
In principle, an operating microscope (Fig. 4.20) has an optical ray path similar to that of a slit lamp microscope (Fig. 4.15). Several requirements are comparable, such as those of a free working area, depth of focus, variable enlargement, and a stereo effect. Unlike the slit lamp, though, the illumination does not have to be freely movable and, for this reason, the light source is firmly built into the microscope. A change between focal and confocal illumination is possible.
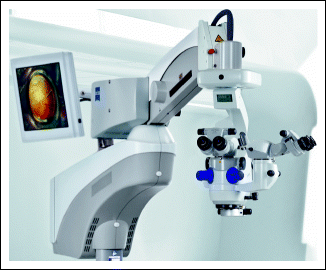
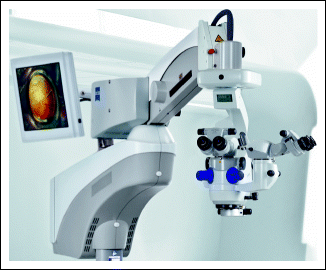
Fig. 4.20
Example of an operating microscope (Courtesy of Carl Zeiss AG, Switzerland)
For the anterior segment, an operating microscope is ideally suited. A very interesting addition, the BIOM,9 together with a SDI works according to the principle of indirect ophthalmoscopy (Fig. 4.21). It enables non-contact 70°–90° wide-angle observation of the fundus during vitreous retinal surgery. A 60–90 D lens in a BIOM produces a bright, inverted image of the fundus. This is then observed through the operating microscope whereby, though, an SDI is put in between that uprights the image by means of a passive optical system of mirrors and, in addition, exchanges the optical ray paths for the surgeon’s eyes to ensure true stereoscopy.
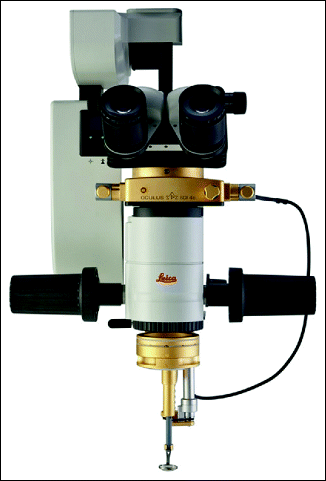
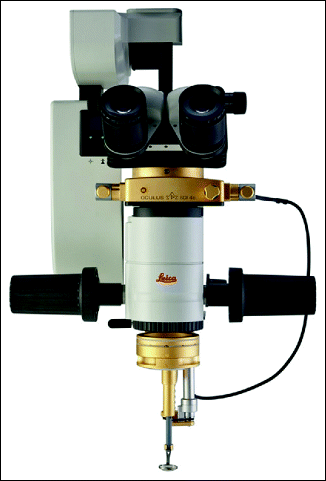
Fig. 4.21
BIOM combined with SDI (Courtesy of Oculus Optikgeräte GmbH, Germany)
4.1.7 Retinoscopy (Skiascopy, Shadow Test)
Retinoscopy is a method for objectively determining the refractive error of an eye (Fig. 4.22). Only minimal equipment is required to obtain the optimal information concerning the refractive status of an eye. The retinoscope (or skiascope10) shines a narrow streak of light onto the retina and the position of the light can then be shifted by slightly rotating the instrument (Fig. 4.23). The physician observes the light phenomena in the patient’s pupil during this motion. With retinoscopy, one attempts to determine the correction for distance. To avoid errors due to accommodation of the patient, accommodation is usually temporarily blocked by the use of cycloplegic eye drops.
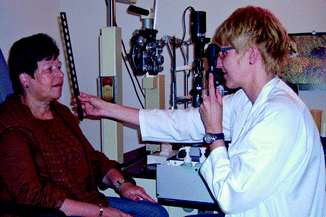
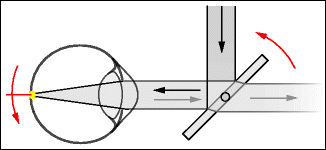
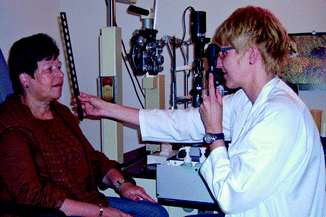
Fig. 4.22
Objective determination of the refractive error with a retinoscope
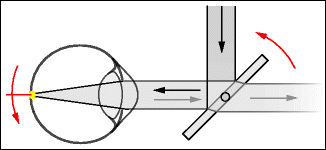
Fig. 4.23
The retinoscope creates a streak of light on the patient’s retina that serves as a secondary light source. With small rotational motions of the retinoscope, the streak moves sideways on the retina. In this figure, the streak stands perpendicular to the diagram plane. The mirror is semi-transparent, making it possible for the physician to observe the appearance of the light inside the patient’s pupil
When the retinoscope sends parallel light into the patient’s eye, an illuminated streak appears on the retina and, in cases of ametropia, it is a somewhat wider streak. This broadening is not essential for understanding retinoscopy. Here, the method will be discussed with the simplifying assumption that a streak-shaped secondary light source exists and moves on the patient’s retina when rotating the retinoscope (Fig. 4.23). The light phenomena seen inside the patient’s pupil depend on the refractive error (see Fig. 4.24):
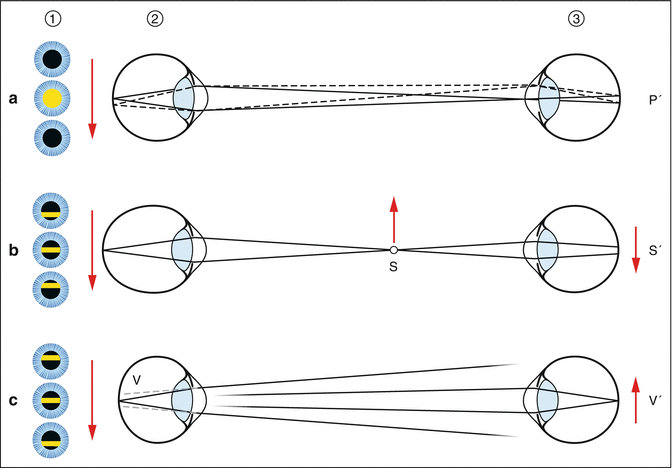
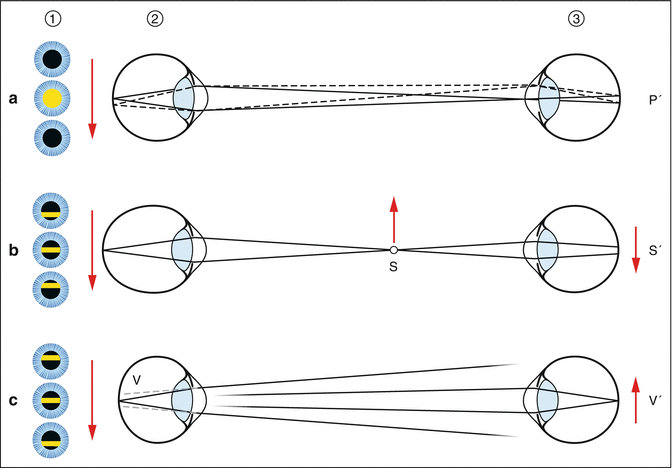
Fig. 4.24
Retinoscopy. Patient (2); observer (3). Situation a: The patient’s eye is focused on the physician’s eye. Situation b: The patient’s eye is focused at a point between himself and the physician. Situation c: the regredient beam is divergent or the patient fixates at a point behind the physician. Column 1 indicates which light phenomenon the physician sees in the patient’s pupil when the retinoscope rotates in the same way as in Fig. 4.23. In situation b, the movement is in the opposite direction, while in situation c, it is in the same direction. P′ Image of patient’s pupil; S′ image of S; V′ image of V. V Virtual image of the light streak on the patient’s retina (V is behind the retina)
A.
If the patient’s eye is focused to the distance of the examiner, a quick change between a bright and dark pupil can be observed in the patient. This is similar to a “flashing” movement, which is also known as the neutralization endpoint (Fig. 4.24a). If the patient is in a cycloplegic state, where accommodation is impossible, the distance refraction can be determined. If the examiner performs retinoscopy from a distance of 50 cm, a 2 D difference exists between the lenses used for neutralization of the reflex and the patient’s true distance refraction.
B.
If the patient’s far point is located between patient and physician (as in cases of higher myopia), a glowing streak is seen inside the patient’s pupil and it moves in a direction opposite to that of the retinoscope (“against motion”; Fig. 4.24b). The streak is narrow when the refractive error is large. A broader streak near the ideal correction is no longer perceived as a streak but as a dark-bright-dark alteration. The point of optical neutralization has then been achieved.
C.
If the patient is hyperopic, the same images appear in the pupil as in (B) but with the inverse direction of motion; that is, the streak in the pupil moves in the same direction as the illumination (“with motion”; Fig. 4.24c).
The phenomena observed by the physician inside the patient’s pupil can be understood in the following way (Fig. 4.24). It is assumed that the physician focuses onto the patient’s pupillary plane:
A.
The easiest case is when the patient focuses on the physician’s pupil. Independent of the position of the light source, the physician always sees the same image: the glowing pupil of the patient. The optical ray path indicates that the image P’ of the patient’s pupil does not move on the retina of the observer while the light streak moves on the patient’s retina. However, the patient’s pupil switches to dark when the focused light is next to the physician’s pupil. Therefore, an abrupt change is noted between the dark and fully illuminated pupil of the patient (“blinking”).
B.
The glowing streak on the patient’s retina is seen to be at a location S, between the physician and the patient, and it functions there as a tertiary source of light. The physician sees its image S′ as blurred or the streak as widened. The movement of S is opposite to that of the retinoscope.
C.
In hyperopia, the light beam leaves the pupil divergently. The observer then sees the backward extension of this beam as a virtual light source V. It moves in the same direction as the light streak on the patient’s retina. (This is also true when the patient fixates on a point behind the head of the observer or at infinity. This situation is not depicted in the figure.)
So far, we have discussed the so-called streak retinoscopy. In contrast to spot retinoscopy, it has the advantage that the determination of astigmatic corrections is easier. The figures do not indicate a situation where the streak projected onto the retina by the retinoscope may be blurred. This is of subordinate importance because we have been concerned here with discerning movement directions.
4.1.8 Refractometry
Like retinoscopes, refractometers objectively determine an eye’s refractive status. A special target is projected onto the retina and the refractive error of the eye is then judged based on the retinal image. The examiner observes this image through optics with a small aperture to circumvent aberrations of the examined eye. The lenses required to see the target in focus correspond to the patient’s refractive error. The Raubitschek chart is often used as the test target (Fig. 4.25).
< div class='tao-gold-member'>
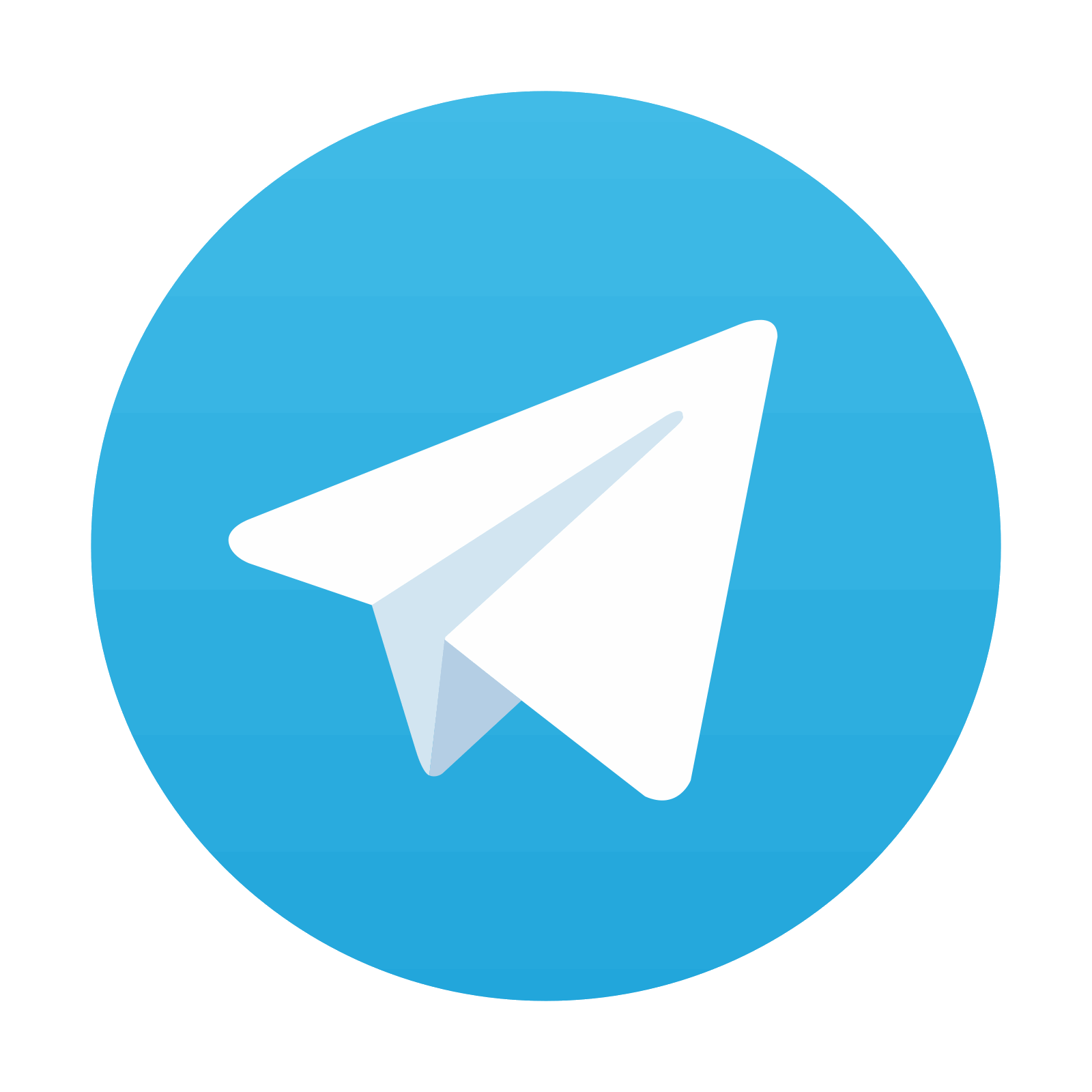
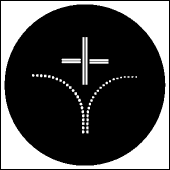
Only gold members can continue reading. Log In or Register a > to continue
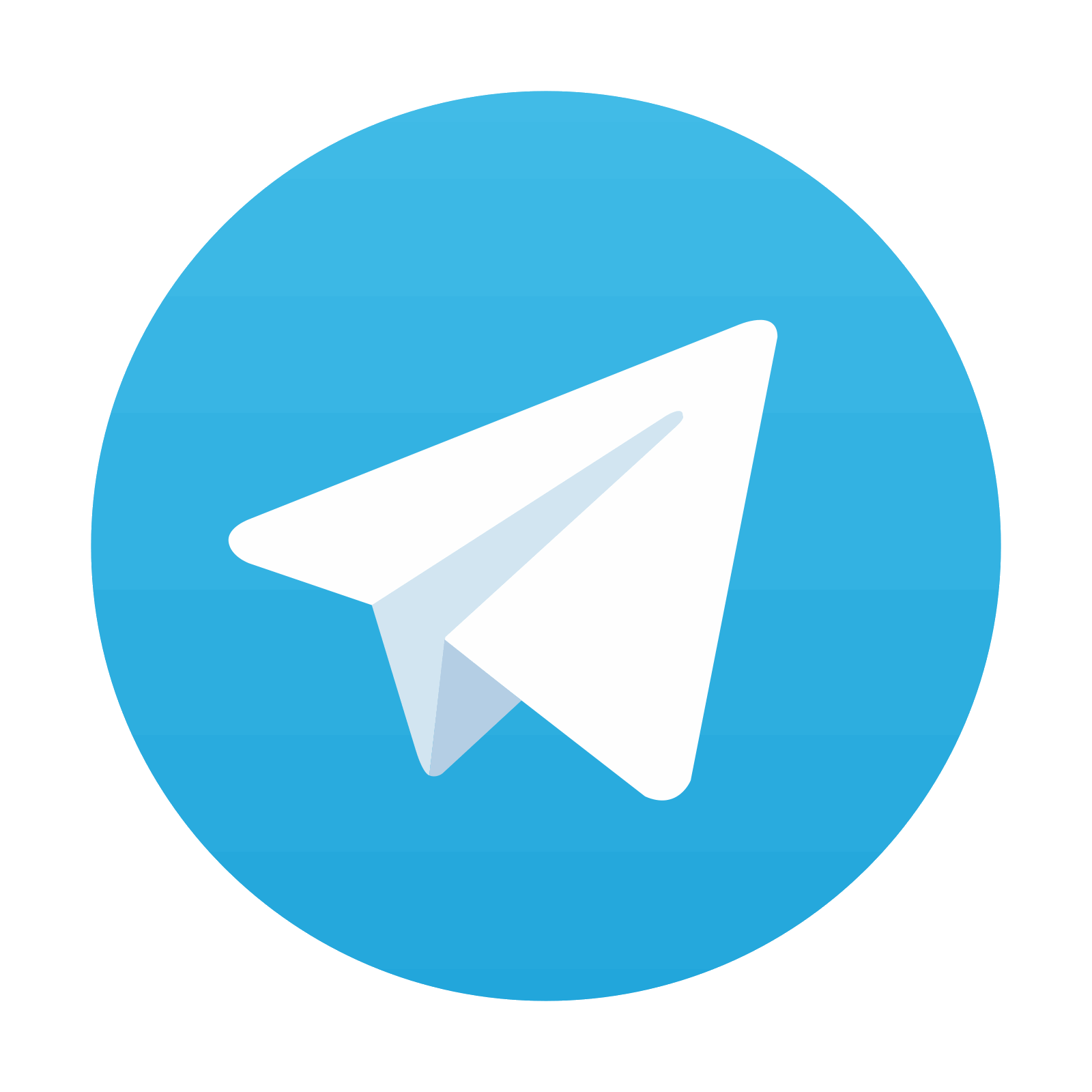
Stay updated, free articles. Join our Telegram channel
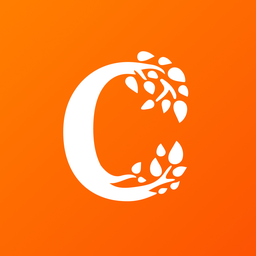
Full access? Get Clinical Tree
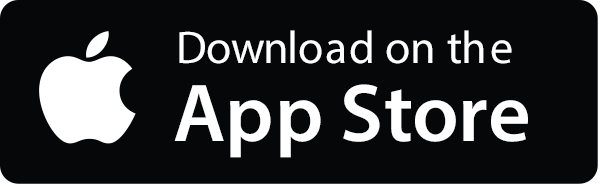
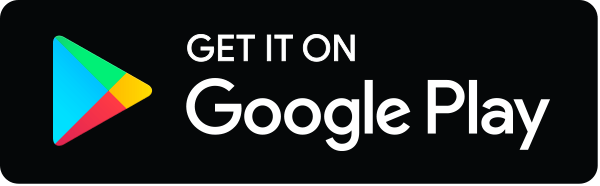
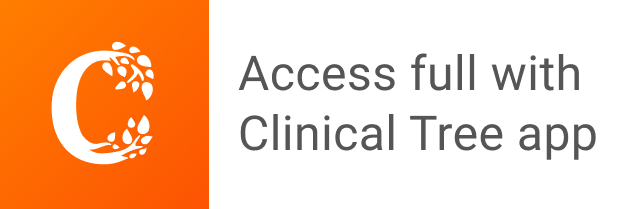