Introduction
The purpose of this chapter is to review a number of the clinical instruments that are of utility in the preliminary examination and ongoing care of contact lens patients. The emphasis will be on the design and principles of operation, with some comments on clinical use. Further details on the application of these instruments in contact lens practice can be found in Chapter 38 . The instruments discussed here form only a subset of the full range of instruments that should be available to eye care practitioners (ECPs) for examining all aspects of ocular health and visual function. General ophthalmic instruments that are used frequently (e.g. refracting equipment, retinoscopes, ophthalmoscopes) and periodically (e.g. tonometers, visual field analysers, colour vision and binocular vision assessment apparatus) in the course of a contact lens examination will not be considered here.
Observation of the Eye
Perhaps the most fundamental procedure in a contact lens consultation is the examination of the anterior ocular structures. The standard technique for examining the eye in detail is slit-lamp biomicroscopy, which has been available to practitioners since the invention of contact lenses over a century ago. More recently, high-powered observation tools have become available that allow examination of the living cornea at a cellular level.
Burton Lamp
A number of manufacturers make a handheld magnifying device for contact lens work. This device is usually referred to as a ‘Burton lamp’, after the company that manufactured the original version (Burton Manufacturing Co., United States). The Burton lamp is essentially a large magnifying lens of about +5.00 D housed in a broad frame, within which are mounted a combination of 4-W white light and ultraviolet light fluorescent tubes, each 11-cm long. The operator can switch between the two light sources for white light and fluorescein examinations. A key advantage of this instrument is that both eyes of the patient can be viewed simultaneously, facilitating interocular comparisons, which can be particularly useful in the course of fitting both rigid corneal and scleral lenses ( Fig. 34.1 ).

Examination of the Tear Film
A ‘healthy’ tear film is essential for successful contact lens wear, and a number of techniques are available for its assessment.
Tear Quality and Thickness
The Tearscope-plus (Keeler, United Kingdom) can be used to observe certain characteristics of the tear film noninvasively ( ) ( Fig. 34.2A ). This instrument takes the form of a small white dome with a central sight hole, surrounded by a cold light source. It can be held directly in front of the eye, or used in conjunction with a slit-lamp biomicroscope to gain more magnification ( Fig. 34.2B ). The thickness distribution, quality and freedom of movement of the tears can be assessed by observing the reflected light from the featureless white dome, and the integrity of the aqueous and lipid phases can be inferred from colour fringe interference patterns ( ). Although the Tearscope-plus is no longer manufactured, similar instruments have become available more recently. The EASYTEAR View+ (EASYTEAR, Trento, Italy) is designed and functions in a very similar way to the Tearscope-plus and uses white light emitting diodes to permit the user to observe the dynamic interference patterns of the lipid layer and can be used to determine the break-up time of the tear film by inserting a grid pattern ( Fig. 34.3A and B ) ( ). It can be used as a handheld device or mounted onto a slit lamp, which allows the user access to a greater range of magnification to view the features of interest. The Polaris Tear-Film Analyzer (CSO, Firenze, Italy) is an accessory that can be mounted to a slit lamp that also permits interferometric examination of the lipid layer using an LED cold light source from the device ( ).


Another instrument that can facilitate qualitative observation of the lipid layer is the OCULUS Keratograph 5M (Oculus, Wetzlar, Germany) ( ). The interference colours of the lipid layer and their structure are made visible using white light illumination and this pattern can be recorded. The thickness of the lipid layer is then assessed based on the structure and colour of this image, without using a dedicated interferometer.
Grading the lipid layer using the instruments described so far is limited to subjective interpretation. This can be difficult for those with limited experience of looking at tear film lipid layers, especially when the lipid layer is thin and colour fringes are less apparent ( ). The TearScience LipiView II ocular surface interferometer (Johnson & Johnson Vision, Jacksonville, Florida, United States) was developed to evaluate the tear film in patients with meibomian gland dysfunction (MGD) and dry eye ( ). This instrument ( Fig. 34.4 ) uses interferometry to noninvasively measure lipid layer thickness between blinks, and gives a quantitative assessment in interferometric colour units. Blink dynamics and partial blinks are assessed, in addition to the change in lipid layer thickness and appearance with each blink. It can be valuable to image the tear film before contact lens fitting, as the presence of MGD could result in reduced comfort and potentially altered deposition during lens wear ( ).

Tear Break-Up
Rapid tear break-up can lead to symptoms of dryness and discomfort in both lens wearers and nonlens wearers. Tear film break-up can be assessed by instilling fluorescein into the eye and timing how long it takes for breaks in the even, fluorescent glow to appear. The problem with this fluorescein break-up time approach is that it is ‘invasive’, in that the instillation of fluorescein in itself alters the quality and quantity of the tear film ( ).
The preferred approach is to determine the noninvasive tear film break-up time (NIBUT). This can be achieved by optically projecting a grid pattern on to the cornea and timing how long it takes for the grid to become disrupted. Numerous ‘specialist’ devices employing this principle have been produced ( ), including the instrument stand-mounted ‘NIBUT dome’ or ‘Mengher grid’ ( ) and handheld devices such as the keratometer-mounted Hir-Cal grid ( ), the Loveridge grid ( ) and the Tearscope-plus and EASYTEAR View+ using their respective NIBUT grid attachments ( ). One option to observe the tear film break-up without purchasing a dedicated device is to project the placido rings from a corneal topographer onto the front surface of the cornea and manually count the seconds for the projected grid to distort ( ). Previous attempts have been made to automate this mire distortion ( ). More recently, the Keratograph 5M (OCULUS, Wetzlar, Germany; Fig. 34.5 ) has developed an automated tear film scanning process that allows automatic, examiner independent determination of tear film break-up time, providing a graphic representation referred to as a ‘tear map’, which shows the location of the tear break-up ( ). Colour coding indicates the regions of break-up: green indicates a stable tear film, yellow indicates a thinning tear film and red indicates tear film regions that are unstable ( Fig. 34.6 ).


Osmolarity Assessment
A clinical test that is reportedly highly diagnostic involves measuring tear film osmolality ( ). This is often considered a ‘gold standard’ in the evaluation of patients with dry eye ( ), due to the hypertonic tear film found in dry-eyed individuals ( ). A hypertonic tear film causes ocular surface damage and may lead to discomfort ( ). Even though tear osmolality has been considered a valuable method for diagnosing dry-eye syndrome for several decades, until recently it has not been used widely as a clinical tool due to the lack of available equipment and the fact that most osmometers require a large volume of tears (typically 5–10 µL) ( ), which limits its use in many dry-eye subjects, particularly those with severe disease in which tear volume is very low.
Historically, measuring tear film osmolality was undertaken using a freezing-point depression method ( ), most frequently using the Clifton instrument. However, this instrument requires considerable expertise, is time-consuming, and the equipment is difficult to maintain ( ). More recently new devices have become available that require far less tear fluid and are easier to operate.
TearLab
This so-called ‘lab-on-a-chip’-based osmometer (TearLab: TearLab Corporation, San Diego, California, United States) ( Fig. 34.7 ) uses electrical impedance and requires only 50 nl of tear fluid ( ). A test card is inserted into a ‘pen-shaped’ holder, which is gently placed in contact with the tear film located in the lower tear meniscus for approximately 30 seconds. This is then placed into a portable countertop unit that calculates and displays the osmolarity test result. The reader automatically converts the tear fluid sample data into an osmolarity measurement and displays the reading on the LCD display. This rapid acquisition of osmolality has resulted in an expansion of the utility of osmolality determination in clinical practice ( ). In addition, the adoption of osmolarity as a method to diagnose dry eye disease ( ) has resulted in increased interest in the assessment of osmolarity in patients, including those who are contact lens wearers.

I-Pen
The I-Pen osmometer (I-MED Pharma Inc, Quebec, Canada) measures tear osmolarity by measuring the electrical impedance of the extracellular fluid contained within the palpebral conjunctiva ( ). It consists of a disposable Single Use Sensor (SUS), which is inserted into a handheld pen-like device. Patients are asked to gently squeeze their eyelids closed for 30–60 seconds. Patients then open their eyes, and the tip of the SUS is placed at a 30- to 45-degree angle directly onto the palpebral conjunctiva on the inside of the retracted lower eyelid, with the gold node from the SUS in good contact with the palpebral conjunctiva. After a few seconds in this position, the handheld osmolarity system makes an audible beep and displays the osmolarity reading in milliosmole per litre on its LCD screen ( ).
Tear Meniscus Height
An adequate volume of tears is a prerequisite for a healthy ocular surface ( ) and a reduction in the volume of tears gives rise to a greater chance of symptoms of ocular dryness ( ). Estimation of tear volume is often undertaken using Schirmer strips ( ), phenol red threads ( ) or estimating the volume of tears in the inferior tear meniscus ( ). The inferior tear meniscus contains about 90% of the tear volume ( ), and tear meniscus volume is classically determined by estimating the tear meniscus height (TMH) ( ). Studies have shown that TMH estimation is a good clinical indicator in identifying aqueous deficient dry-eyed patients, as it is a direct measure of the quantity of the tear film ( ).
The most common and simple method to determine TMH is visualizing the TMH using a slit-lamp biomicroscope with an eyepiece containing a graticule ( ). However, estimation of the upper border of the TMH is difficult and several studies have achieved this by adding a small volume of fluorescein to the tears ( ). Enhancing the visualization of the upper TMH border by the addition of fluorescein is clearly invasive and may interfere with the tear volume determined, resulting in an overestimation of the TMH.
To overcome such difficulties, a number of noninvasive approaches to measure TMH have evolved, including videography of the meniscus ( ), optical pachymetry ( ) and meniscometry ( ).
Various multifunction devices now exist that can routinely photograph the TMH and include built-in software to determine the TMH. These instruments typically include the measurement of TMH as part of a battery of tear film assessments, such as noninvasive tear-break-up time and meibography, aimed at measuring signs related to various aspects of dry eye disease. Many of these devices allow the user to photograph the lower TMH and use a ruler built into the software to determine the height of the tear meniscus ( Fig. 34.8 ) ( ).

Optical coherence tomography (OCT) has been used to accurately determine TMH ( ). Once the tear prism is visualized, images can be scanned and stored and the height within the OCT slice is calculated ( Fig. 34.9 ). OCT offers an advantage in that it is the only available method to view the tear prism in cross-section to determine cross-sectional area (allowing for tear volume calculation) ( ) and can also be used to determine the TMH of the upper lid ( ).

Examination of the Meibomian Glands
A variety of consensus-based workshops conducted by the Tear Film and Ocular Surface Society (TFOS) all emphasize the importance of having adequately functioning meibomian glands for optimal ocular surface health and contact lens comfort ( ). ECPs can simply evaluate the meibomian glands by merely applying firm, digital pressure to the lower lids, close to the lid margin, and observing the meibomian gland orifice for appropriate meibum expression. However, there is inherent variability in this methodology and a number of handheld tools are now available that can be used to evaluate meibomian gland expressibility and meibum quality. These tools all apply pressure to the lid margin and are often used with the patient positioned behind the slit lamp to observe the meibomian gland orifices under greater magnification. The examiner typically assesses all glands along the length of the lid margin.
The Mastrota paddle (OcuSOFT, Rosenberg, Texas, United States) ( Fig. 34.10 ) is designed to gently and effectively express meibum from the meibomian glands ( ). It is positioned between the eyelid and the eye, parallel to the glands while a finger or cotton-tip applicator applies gentle pressure to the outer lid. This procedure can be carried out with or without anaesthesia. While this procedure requires the use of two hands, other tools such as the Collins Gland Expressor Forceps (Bruder Healthcare Company LLC, Georgia, United States), Tearse Meibum Expressor (Rhein Medical Inc, Florida, United States) and Bruder Roller Expressor (Bruder Healthcare Company LLC, Georgia, United States) allow for single-handed evaluation of the meibomian glands. The eyelids are placed between the forceps of these instruments and the examiner applies pressure to squeeze the lids and observes the meibum expressed from the glands. As with digital expression and expression using the Mastrota paddles, the use of forceps for gland expression delivers variable force to the lid margins between assessments and there is a risk that excessive force is used, such that the procedure becomes a therapeutic technique to evacuate the glands, rather than a diagnostic technique.

The handheld Meibomian Gland Evaluator (Johnson & Johnson Vision, Jacksonville, Florida, United States) ( ) offers the advantage of standardizing this technique, regulating the consistency of pressure at 1.25 g/mm 2 ( Fig. 34.11 ). When used correctly, the applied level of pressure is equivalent to a blink, therefore the practitioner can observe how much meibum is approximately delivered to the ocular surface using this method of assessment. Five glands are assessed at a time and assessments are made in the temporal, central and nasal region of the lower lid of both eyes.

One of the most effective ways of evaluating the meibomian glands is to visualize and photograph their appearance using meibography ( ). This method of assessment uses infrared (IR) illumination to determine the number of partial glands or total meibomian gland drop-out ( ). An increasing number of noninvasive, clinician-friendly devices have become available, making meibography a routine procedure within clinical practice ( ). These devices typically feature meibography as part of a suite of other dry eye tests such as noninvasive tear break-up time and TMH assessment. Examples of these instruments include the Keratograph 5 M (OCULUS, Wetzlar, Germany) ( ) ( Fig. 34.12 ), the LipiView II (Johnson & Johnson Vision, Jacksonville, Florida, United States) and the Medmont Meridia (Medmont International Pty Ltd., Victoria, Australia). One model of the slit lamp-mountable EASYTEAR View+ (EASYTEAR, Trento, Italy) features built-in IR LEDs, which, when used with a digital camera sensitive to IR wavelengths, permits the imaging of meibomian glands without having to move away from the slit lamp ( ).

Multifunctional Instruments for the Assessment of the Ocular Surface
Instruments that serve several functions are becoming increasingly powerful and appeal to clinics that specialize in a particular area of clinical focus or prefer to maintain one device that serves many purposes, to maximize use of space. Over the last decade, many multifunctional instruments capable of assessing multiple ocular surface features have become commercially available. The development and availability of these instruments have largely been driven by the publication of several seminal workshops, particularly the TFOS Dry Eye Workshop II (DEWS II) Diagnostic Report ( ), and a shift towards evidence-based practice.
Many of the features of multifunctional instruments that assess the ocular surface utilize a placido-based system, thus are corneal topographers with additional ocular surface assessment capabilities. Differences in the algorithms used in the accompanying software exist, but some of the most common ocular surface assessment features include:
- •
Noninvasive tear break-up time
- •
TMH measurement
- •
Imaging capabilities (video or still image) using white light or blue light, including determination of ocular redness
- •
Built-in IR illumination for meibography or nonwhite light assessments of tear break-up time and TMH.
Table 34.1 summarizes several multifunctional instruments that focus on ocular surface assessments. Some software provided by the manufacturers of these instruments allow practitioners to enter the user’s subjective grading of the features observed using the instrument and enter data collected from instruments collected using the instrument (e.g. osmometry), to generate a full dry eye diagnostic report.
One of the earliest developments within these multifunctional devices was the objective assessment of ocular redness. In clinical practice, ocular findings are typically assessed subjectively by means of image-based grading scales ( ). Despite the obvious convenience of their use, the inter- and intra-observer variability between repeated assessments has been open to criticism ( ). To overcome this limitation, various objective assessment methods have been proposed, including image analysis ( ) and photometric measurements ( ). Despite the advantages of these techniques, they are not easily achievable in clinical practice and remain largely used within research studies.
The Keratograph 5M (OCULUS, Wetzlar, Germany) uses modified optics to image the patient’s cornea and also the visible nasal and temporal bulbar and limbal conjunctiva while the patient is looking straight ahead at the centre of the Placido disc rings ( ). The curved Keratograph head, with its concentric Placido disc rings, allows for consistent and uniform illumination across the visible conjunctiva ( ). The built-in software automatically detects the visible nasal and temporal conjunctiva, and estimates redness by quantifying the area covered by vessels relative to the overall detected area ( ). The Keratograph 5M output provides bulbar as well as limbal redness grades on a 0 (none) to 4 (severe) scale, in 0.1 increments, for both the nasal and temporal conjunctiva. In addition, an overall bulbar redness score based on a weighted average of nasal and temporal redness and the analysed area (in mm 2 ) are displayed ( Fig. 34.13 ) ( ).

The Keratograph 5M allows a direct comparison of the acquired image to a published grading scale (the JENVIS 0–4 scale) ( ), which can help facilitate communication of the results with patients ( ). The hyperaemia results using this technology appear to be highly correlated to subjective grading ( ), have less variability than subjective assessments obtained by means of traditional grading scales ( ) and can be used to assess redness in patients with ocular dryness, allergies and contact lens wearers ( ).
Some multifunction devices, such as the Topcon MYAH (Topcon Healthcare, Oakland, New Jersey, United States), share the same ocular surface modules described in other multifunctional instruments but also include features required in monitoring the progression of myopia and effectiveness of myopia control strategies, such as axial biometry and pupillometry.
Slit-Lamp Biomicroscopy
The slit-lamp biomicroscope plays an essential role in the preliminary assessment and aftercare of the prospective and existing contact lens wearer. The opportunities for using the slit lamp within the routine eye examination are numerous and diverse. With the appropriate application of supplementary lenses and/or viewing techniques the instrument may be used to assess the condition of the vitreous, lens and retina from posterior pole to the ora serrata. Various ancillary instruments will permit examination of the anterior-chamber angle, measurement of intraocular pressure, corneal sensitivity and assessment of corneal thickness. This review will concentrate on the instrument itself: see Chapter 35 for an account of the use of the slit-lamp biomicroscope in contact lens practice.
The instrument consists of a separate illumination system (the slit lamp) and viewing system (the biomicroscope), which have a common focal point and centre of rotation ( Fig. 34.14 ). A height control moves both systems simultaneously, while focusing and lateral movements are achieved via a joystick. This common control feature facilitates rapid and accurate positioning of the slit beam on the area of interest and ensures that the microscope and illumination system are simultaneously in focus.

Illumination System
Virtually all slit-lamp manufacturers have adopted the Koeller illumination system, which is optically almost identical to that of a 35-mm slide projector ( ). A bright illumination system (producing approximately 600,000 lx) is a fundamental requirement for a slit lamp if subtle conditions are to be seen clearly. While tungsten lamps have been the most frequently used illumination sources in the past, halogen and more recently LED illumination sources have now taken over. Illumination sources of currently available slit lamps from manufacturers such as Zeiss or Haag-Streit exclusively rely on halogen and LED lighting ( ). The recently introduced Zeiss SL 800 offers a combination of an LED light source that is accompanied by a halogen filter, termed VarioLight, that is intended to provide the clinician with the benefits of both kinds of illumination sources ( ).
Haag-Streit incorporated halogen background illumination as a secondary light source to some slit-lamp models (e.g. standard in BX 900 or optional for BI 900, BP 900 or BQ 900), to allow for more uniform illumination when imaging clinical findings ( ). Illumination brightness is controlled by a rheostat or multi-position switch, such that brightness can be adjusted to obtain the correct balance between patient comfort and optimal visibility of the area of interest.
To allow for appropriate illumination for a variety of clinical conditions, the illumination system of a slit lamp should include the following features:
- •
Sharply demarcated edges of the slit within the illumination system.
- •
An adjustable slit width and height such that any shaped patch from a slit to a circle may be projected, as this will increase the variety of illumination methods possible.
- •
A graduated slit width, which is particularly useful when measuring the size of a lesion.
- •
An ability to rotate the lamp housing such that the slit may be used in meridians away from the vertical is useful, particularly if a protractor scale is included. Such a system enables, for example, the angle of rotation of a soft toric lens away from the vertical to be accurately measured.
- •
The slit beam must have the capability to be displaced or offset sideways (‘decoupled’). This ability to break the linkage between the illumination and observation systems facilitates indirect illumination techniques.
A number of filters can be incorporated into the illumination system; these serve to enhance the visibility of certain conditions:
- •
Green (‘red-free’) filter – enhances contrast when looking for corneal and iris vascularization, since red vessels appear black if viewed through such a filter. In addition, it may be used to increase the visibility of rose bengal staining on both the cornea and conjunctiva.
- •
Neutral-density filter – reduces beam brightness and increases comfort for the patient.
- •
Polarizing filter – reduces unwanted specular reflections and can be useful to enhance the visibility of subtle defects.
- •
Diffusing filter – diffuses the illumination source over a wide area and is used to provide broad, unfocused illumination for low-magnification viewing of the general ocular surface.
- •
Cobalt blue filter – provides a suitable means of exciting sodium fluorescein for examination of ocular surface integrity. Illumination of fluorescein with cobalt blue light of 460–490 nm produces a greenish light of maximum emission 520 nm. Any abraded area will absorb fluorescein and display a fluorescent green area against a general blue background. The filter is occasionally used on its own to aid in the diagnosis of keratoconus. A frequent finding in this corneal ectasia is Fleischer’s ring, which is formed by an annular iron deposition within the stroma at the base of the cone. The iron pigment is often difficult to see in white light but will usually appear in greater contrast when viewed through the cobalt blue filter.
- •
Kodak Wratten number 12 (yellow) filter – this filter is not contained within the illumination system but is used as a supplementary barrier filter that is placed in front of the viewing system. It significantly enhances the contrast of any fluorescent staining observed with the cobalt blue filter as it allows transmission of the green fluorescent light but blocks the blue light reflected from the corneal surface ( ). Many newer slit lamps incorporate it within the viewing system, but many practitioners use it as an ‘add-on’ device that is placed over the observation lens when required. Custom-made barrier filters for certain slit lamps are available from some manufacturers ( Fig. 34.15 ). Inexpensive handheld versions may be constructed by using a cardboard mask and Lee filters number 101 yellow.
Fig. 34.15
Custom-designed barrier filter in position on the Nikon FS3 slit-lamp microscope. The slider for the zoom magnification system is also clearly seen.
The Microscope
A key prerequisite for a slit-lamp biomicroscope is a viewing system that provides a clear image of the eye and has sufficient magnification for the practitioner to view all structures of interest. Magnification is an important consideration, and ideally magnifications of up to 40× should be possible; this may be achieved through interchangeable eyepieces and/or variable magnification of the slit-lamp objective ( ). More recently, the addition of digital imaging modules and subsequent image analysis has resulted in magnifications of up to 200× being possible ( ), allowing very fine details of the cornea and other external structures to be visible. Magnification greater than this level is usually unnecessary and is often counterproductive, as small involuntary eye movements will render the image too unstable to view. Ideally the practitioner should be able to change magnification swiftly and easily, which gives slit lamps with three or more objectives an advantage. Zoom systems have the added advantage of allowing the practitioner to focus on a particular structure without losing sight of it during changes in magnification. Of course, the magnified image must also be clear, and the importance of choosing a slit lamp with a high-quality optical system cannot be overemphasized. Ideally the microscope should have excellent resolution and a good depth of field. However, these factors are inversely linked and so a compromise must be accepted.
Mastering all of the possible illumination techniques with the slit lamp is essential if the instrument is to be used to its full potential. Practice with the instrument is critical to becoming comfortable with its subtle but extensive variety of uses. Readers are advised to consult other texts for information concerning the use of the many and varied illumination techniques available with the slit lamp ( ).
Digital Imaging
The most common method for recording contact lens fittings or ocular changes remains the use of handwritten (or electronic) notes and diagrams. In recent years these have been improved with the advent of formal grading scales ( ) (see Chapter 38 and Appendix K ). The use of digital imaging complements any written record keeping by providing a visual representation of the recorded clinical finding. In contact lens practice, digital imaging is most often used to document any ocular pathology observed prior to initial fitting or during subsequent follow-up checks.
As with all forms of technology, the cameras and computer systems continue to improve in quality, become less expensive to set up and easier to operate, resulting in more widespread adoption by clinicians. Initial photo-documenting systems used 35-mm film-based cameras and required a slit lamp with a built-in flash tube or a video camera mounted on a slit lamp ( ). One major recent advance relates to the potential use of personal smartphones to capture images ( ), which has substantially changed the digital imaging and recording landscape for ECPs.
Benefits of Digital Imaging for Contact Lens Practice
There are numerous features and benefits of using digital imaging ( ), including:
- •
Instant imaging – the record is simply and instantly made.
- •
Minimal costs – once a digital imaging system is set up, the unit cost for each image is virtually zero. With modern computers having terabyte-sized internal or external storage capacity, many thousands of images may be easily stored and retrieved.
- •
Image manipulation and quantification – after an image is captured, the brightness, contrast or colour may be enhanced. Furthermore, image parameters can be quantified by the computer (e.g. blood vessel length, scar dimensions and cup / disc ratio) or graded using a validated grading system. However, care must be taken not to alter an image that may be legal evidence.
- •
Video movies – dynamic conditions such as contact lens fits or certain dynamic forms of pathology evaluation can be captured as a short movie on the computer ( ). Lens performance is much easier to understand and interpret when a moving (versus static) image is presented.
- •
Portability and accessibility– images can be easily transferred and viewed on smartphones. Some patients love to post their images on social media sites like Facebook or Instagram.
- •
Once the digital image has been captured in an electronic format, this opens up the following possibilities:
- •
Improved patient care – one problem with written notes and drawn diagrams is variability between observers. Even within the same observer, over a long interval between exams, some interpretation of the notes may be required. Photo documentation is valuable to evaluate progression or the impact of a treatment intervention in the patient’s condition.
- •
Patient education – there is a benefit in the patient immediately seeing their own condition. The patient ‘wow’ factor is also beneficial to practice growth, and this may be the biggest pay-off from imaging ( ).
- •
Paperless office – the majority of contact lens practices use electronic medical records for patient visits. Digital imaging is a logical adjunct to electronic records and has great value in cases of potential legal action ( ). With the internet and secured cloud-based storage solutions, patient records can be accessed at more than one office location.
- •
Image transfer – increasingly, clinicians are communicating by email or in virtual meetings. A digital image is already on the computer and this allows for simple sharing with colleagues and patients, and has made consultations with colleagues from all over the world simple, affordable and fast.
- •
Presentations and publications – high-quality digital images and videos can be easily included in presentations, publications or patient handouts.
In summary, photo documentation enhances communication with patients as well as improving the quality of clinical records. With the increased use of electronic medical records, hand-drawing is reduced, making digital imaging more necessary ( ). The merits of photo documentation are numerous and digital imaging has taken clinical documentation to another level.
Resolution Requirements for Anterior Digital Imaging
The basic principle of digital imaging is that a light-sensitive silicon computer chip is used in a camera. The silicon chip is known as a charge-coupled device (CCD), and the number of light-sensitive elements on the CCD determines the image resolution for video and digital cameras. The most frequently used types for slit lamp-based digital imaging are digital single lens reflex (SLR) cameras, video slit-lamp imaging systems using a wide variety of devices and, increasingly, smartphone cameras. Modern smartphone cameras can take good-quality high-resolution images through the eyepiece of a slit lamp at varying illumination levels ( ).
The image resolution refers to the image dimensions (width × height) in units of the number of dots (pixels). As the image proportions for most cameras are in a 4:3 aspect ratio, the resolutions are simply referred to as a million pixel count (e.g. 10 MP). One way to consider this question of ‘how much resolution is enough’ is by the unit of detail that needs to be recorded. Fig. 34.16 shows a number of clinical signs that an ideal digital imaging system should be able to capture. Examples are: (1) corneal punctate staining, (2) keratic precipitates, (3) endothelial cells and (4) epicapsular stars. These features are 20–50 μm in size and if the digital imaging system can surpass this resolution by a factor of 10 (i.e. 2–5 μm), then the characteristics of each feature should be discernible in the final image. A 15-MP camera on a slit lamp with 16 × magnification should give this resolution (i.e. 3 μm). Ye and colleagues ( ) measured the spatial resolution of a commercial slit lamp with an SLR camera to be near 2 μm, although they commented that the spatial resolution apparent to the observer was higher still.

Improving Image Brightness
There are four commonly used illumination techniques for photo documentation:
- •
Diffuser – this is best for opaque tissues, such as the conjunctiva, sclera and eyelids. It is used in conjunction with a full-width slit, and the brightness of the beam is varied for optimum exposure.
- •
Slit section – this is most useful for transparent tissues. An angled slit beam gives a dark-field background to highlight features. A slit section can also be used with retroillumination so as to highlight opaque tissue changes.
- •
Fluorescein staining – surface defects are best shown with the combination of a cobalt blue illumination filter and a yellow barrier filter with a wide slit beam ( ).
- •
Tear film assessment – specular illumination with the slit lamp or other instrument will show lipid layer patterns, tear film thickness and break-up ( ).
Underexposure of anterior eye digital images is a common problem. The camera needs to have good low-light performance to obtain high-contrast slit images or to show fluorescein staining with filters in place. Smartphone cameras are typically fully automatic and obtaining an image with the slit lamp on a high light level at a low magnification will often provide images at an appropriate illumination level. In certain scenarios, the use of external light sources that provide additional, uniform lighting may be of benefit to obtain better images.
A low magnification gives a larger exit pupil for the biomicroscope optics, so more light will enter the camera. Where possible, turn off the smartphone flash and autofocus. Excellent results can be obtained with a digital SLR camera. For a modern digital SLR camera, it is preferable to apply the manual settings (e.g. a high ISO speed of 1600 or more and a slower shutter speed of 1/15th to 1/25th of a second). A full-featured digital slit lamp has a flash system built in to the illumination path; however, such slit lamps carry a higher cost and are more complex.
Examples of digital images for each of the illumination types are shown in Fig. 34.17 .

Commercial Digital Imaging Systems
Contact lens practitioners wishing to commence digital imaging in their practice have the choice of purchasing a commercially produced system or getting started using a smartphone camera via a dedicated adaptor. A commercially produced digital imaging system can be defined as a ready-to-use integrated system, sold by an ophthalmic equipment supplier. It will typically consist of a slit-lamp biomicroscope attached to a video camera or digital still camera, and a computer with a database and image manipulation software.
A commercial digital imaging system is probably the best alternative for most practitioners, as these systems have the benefit of a relatively simple interface and an easy-to-use database for managing records. The company supplying the equipment will have taken care of the technical issues related to setting up image acquisition by the computer from the video camera. Examples of commercially available digital imaging systems include the Haag-Streit BX 900 slit lamp with a digital SLR camera and Xenon flash (Haag-Streit AG, Koeniz, Switzerland), or the Zeiss SL 800 slit lamp in combination with the SL Imaging Solution (Carl Zeiss Meditec AG, Jena, Germany).
The advantage of a digital still camera is in the high quality and resolution of the image. Professional digital SLR cameras afford high resolution (15 million pixels or more), good low-light performance and options for attaching them to various instruments. For example, the Takagi slit-lamp biomicroscope (Takagi-Seiko Co., Nagano-Ken, Japan) can be attached to a Canon EOS 500 D camera (Canon Inc., Tokyo, Japan) or a camera of equivalent specification. Other features of such a system include:
- •
The digital camera adaptor (TD-2A) includes a yellow written filter to give high-contrast fluorescein images of staining or lens fittings.
- •
The camera adaptor places the camera away from the binocular eyepieces, to avoid interference with stereo viewing by the observer.
- •
The slit lamp has an integrated trigger button included on top of the joystick. In this way, photos are taken with the maximum of convenience in normal slit-lamp operation.
- •
An accompanying computer is connected via a USB cable, so that the images taken are instantly viewed and saved. The computer may be directly interfaced with the practice medical record system, for easy integration of file access and connection to the patient record.
- •
A separate background fibreoptic illuminator is included for broad illumination of the ocular and preocular tissues.
A commercial digital imaging slit lamp with an SLR camera has the highest image spatial resolution compared with other slit-lamp imaging methods ( ). Yuan et al. ( ) found the spatial resolution of a Nikon FS-2 slit lamp connected to a Canon 60 D digital SLR camera (18 MP) was comparable with ultra-high-resolution OCT, but for a fraction of the cost.
A video slit lamp may be a relatively low-cost method to capture an image, for display on a screen or to input into a computer. It has the advantage over the smartphone method of being a permanent installation, but typically has a lower resolution and quality than a digital SLR camera. The two key steps are to connect a video camera to the slit lamp and to input the video signal into the computer. The permanent connection of a video camera to a slit lamp requires a beam splitter in the slit-lamp observation system.
Some slit lamps, like the Haag-Streit BD900 (Haag-Streit AG, Koeniz, Switzerland), are supplied with a built-in beam splitter. This is a neat design, since it is in line with the observation system and makes it easy to connect a video (CCD) camera. Other slit lamps, such as the Zeiss SL120 or CSO SL990, have a modular design and the beam splitter and camera can be readily added together. Similarly, some manufacturers offer optional digital camera attachments with a built-in beam-splitter, such as the Topcon DC-4 digital camera attachment (Topcon Medical Systems Inc., Oakland, United States), thus allowing for retrofitting many existing slit-lamp models with high-quality video recording options. In general, if a digital video camera is to be added, it is always recommended to check with the slit lamp or video camera supplier as to whether imaging attachments are compatible with an existing slit lamp or if a suitable beam splitter is available for purchase.
For slit lamps that already include or are retrofitted with a beam splitter, a compatible video camera must be selected. In those cases, a single- or three-CCD microcamera with separate power supply is a suitable option. These are known as ‘lipstick’ cameras, owing to their compact size, making them suitable for an ophthalmic instrument. The low-light performance, resolution and colour rendering are acceptable for most slit-lamp applications. If a video camera with a digital (Firewire or USB) output is selected, then it will connect directly to the computer. The image can then be displayed on the computer, saved or printed. This option has the benefits of good low-light performance, live image preview and the facility for digital movie capture.
Smartphone Digital Imaging
The ever-increasing resolution and image quality that can be achieved with the newest smartphone models make a compelling case for choosing smartphones as the imaging solution to retrofit an existing slit lamp. Perhaps the best reasons to consider using a smartphone for digital imaging is the ease of getting started, the low entry cost and the portability – a fraction of the price of a commercial system. Assuming the availability of a smartphone with imaging capabilities, the only costs are an eyepiece adaptor and potentially a beam splitter (if not already available as part of the existing slit-lamp biomicroscope). While custom-made 3D-printed adaptors have been successfully used for smartphone imaging for a few years ( ), more and more smartphone adaptors are also becoming commercially available, such as the MicroREC ClickNFit system (Custom Surgical, Munich, Germany) or the ACCU-Beam UNIVERSAL Smart Phone adaptor (TTI Medical, San Ramon, United States). The MicroREC ClickNFit optical system also includes the dedicated MicroREC App, a mobile application for managing images and videos of microscopy procedures captured using smartphones. Smartphone adaptors are compatible with most slit lamps, including models by Haag-Streit, Zeiss, Topcon or Keeler, and are available for many smartphone models.
As with any digital imaging system, there is a learning curve towards taking good-quality images. Where possible, turn off the smartphone flash and autofocus. Initially the best images will be most readily taken using a uniform illumination of the eye, with a broad beam or diffuser in front of the light beam. Obtaining images of opaque tissues such as eyelids, the conjunctiva, sclera or iris should be straightforward. Lower-light imaging such as obtaining fluorescein images, narrow optic sections or higher magnifications may take more time to determine the optimum settings. Images can be transferred from the phone to the practice computer system, usually using wireless ‘drop boxes’ online or via email. There is also the potential for global telemedicine using a smartphone for real-time slit-lamp eye examination ( ).
Digital imaging applications for smartphones are increasingly being described in the literature. It is possible to quantify pupillary anisocoria using a smartphone as a macro-camera ( ), or to use a smartphone for imaging through a desktop microscope ( ) or for the clinical assessment of ocular pathogens such as Demodex ( ).
Recording Digital Movies
Two important uses for movies include recording the dynamic procedures involved in lens fittings, and showing corneal complications as the light source is moved or scanned. Viewing of fluorescein patterns, lens centration and movement, or the assessment of the lens surface for defects or looking at lens wettability benefits immensely from the use of digital movies. Recording of videos also allows the visualization of meibum quality during meibomian gland expression. Most smartphones, video and digital SLR cameras have included software to allow movie recording and these video files can then be linked to the patient record.
Postprocessing and Analysis
Digital acquisition of images has the additional benefit that a patient’s history and contact lens wear progress can be closely monitored by visual inspection of images and documentation of changes for many ocular conditions. Software packages such as the AOS software (Advanced Ophthalmic Systems, Croydon, United Kingdom) further enhance patient management capabilities in clinical practice. The AOS software provides clinicians with various features to manage and monitor their patients, including objective grading of the anterior segment (AS), image enhancement, patient and clinician mobile imaging apps, live video calling between practitioner and patient, as well as creating patient reports. Digital images of contact lens wear related clinical findings can be imported into the AOS software, and semiautomated objective grades for bulbar conjunctival redness, palpebral redness or corneal fluorescein staining can be obtained. After importing the images, the clinician manually outlines the area of interest, and the software auto-generates an objective grade within a 0–4 scale range with 0.1 increments ( ). A study comparing grades for bulbar and palpebral redness obtained using the AOS software to grades obtained using the Efron and CCLRU grading scales found the automated grades to be highly reproducible ( ). In addition to objective grading, the AOS software also includes features such as built-in red-free or Wratten filters to enhance the images, a ruler to perform areal or linear measurements (e.g. for the assessment of neovascularization), or the option to annotate the images with additional patient information or reported symptoms. The AOS software is also available as smartphone app, which allows patients to use their smartphone camera to acquire an image of their eye that can then be safely shared with the practitioner via the app, to support virtual patient assessments and counselling.
File Back-Up and Printing
Whatever system is used, it is vital to have a means of backing up the data stored on the slit lamp-interfaced computer, for example by using a recordable CD-RW or DVD-RW, a removable USB hard disc or a flash memory drive. Recently, the costs of external hard-drive systems have become very reasonable. Attaching an external multi-terabyte drive is convenient and easy to do. Increasingly, backing up to the cloud is becoming commonplace and this merely requires an internet connection and adequate storage space. If the images obtained need to be printed (typically to give a copy to the patient), then there is a wide range of printers available, each with its unique benefits. For most situations a colour inkjet printer is appealing because of its compact size, low capital cost and high-quality prints.
Image Editing
Digital images have the advantage of image manipulation post capture, and there are many image-editing programs available to undertake this step. Basic image-editing capabilities are offered as a software feature in some commercial slit-lamp packages.
Key editing applications with respect to contact lens imaging include the following:
- •
Brightness and contrast correction – images that are underexposed can be corrected easily with image editing ( Fig. 34.18 ). The lightest pixel and darkest pixel in the picture specify each end of the spectrum. The remaining pixels are rescaled to fill out the rest of the spectrum ( ).
Fig. 34.18
Image enhancement. (A) The original image. (B) The same image after brightness and contrast enhancement.
- •
Fluorescein pattern analysis – fluorescein pattern images can be analysed by colour ( ). Objective methods can be used to determine the fit of a lens (flat, steep, aligned). The fluorescein pattern is analysed by RGB colour channels. The histograms of the blue and green channels can be used to differentiate between a flat, steep and aligned (on-K) fit ( ).
- •
Tear layer thickness analysis – tear layer thickness under the lens can be determined by the intensity of the fluorescence. The thinner tear layers are dark and thicker tear layers are brighter. The corneal topography underneath a rigid corneal lens can be interpolated with this technique ( ).
High-Powered Microscopy
Conventional microscopy collects all the light reflected back through the object. As a result, information out of the focal plane, above and below areas of interest, creates noisy and blurred images in all but the thinnest specimens at high magnification ( ). Over the past 25 years two techniques (specular and confocal microscopy) have emerged that have enabled researchers and clinicians to examine the structure of the cornea in vivo at very high magnification.
Specular Microscopy
The specular microscope allows viewing of objects illuminated from above, and the objective lens also acts as the condenser lens. Light passes from inside the microscope out through the objective lens to arrive at a focus near the focal plane of the lens. If this position coincides with a reflecting surface, then the focused light is reflected back through the objective lens and is viewed through the eyepiece of the microscope ( ). The first specular microscopes used for ophthalmic research were utilized by David Maurice in the 1960s in his work investigating corneal function. This technique enabled high-magnification images of both the epithelium and endothelium to be made, which had previously been difficult due to their transparency. Early versions of the specular microscope used a contact dipping cone objective lens that was optically coupled to the cornea to provide higher magnification and resolution. However, most modern clinical specular microscopes can achieve equally high magnification without the need for ocular contact. These instruments are primarily used to view and photograph the corneal endothelium. The endothelial cells can be imaged because the refractive index of the endothelial cells is higher than that of the aqueous humour. By direct viewing with the specular microscope an overall impression of the condition of the endothelium can be established immediately.
Typically the features looked for are the regularity of the endothelial mosaic, the size of the individual cells, the presence of intracellular vacuoles and abnormal features such as corneal guttae and keratic precipitates ( ). From the images obtained, factors such as the number of cells per unit area, cell shape and cell area can be calculated, enabling the clinician to assess the endothelial appearance compared with that expected of normal age-matched individuals. Results from these investigations ( ) have shown that the endothelial cell population density drops from approximately 4500 cells/m 2 at birth to 2000 cells/mm 2 in old age, and that their shape and size change dramatically over this time. At birth the endothelial mosaic is very regular and the cells are almost circular in shape. With time they become increasingly angular in shape and varied in size, a condition termed ‘polymegethism’ (see Chapter 38 ). In addition to age-related changes, the specular microscope has been used to investigate endothelial changes in a number of disease conditions, including posterior polymorphous dystrophy, Fuchs’ dystrophy, corneal surgery, refractive surgery and contact lens wear ( ). In addition, deep stromal opacities such as glass foreign bodies, pigment deposits and corneal dystrophies can be imaged ( ).
Confocal Microscopy
Confocal microscopy is unlike conventional microscopy because defocus causes the image to disappear rather than appear as a blurred image. The properties of the confocal microscope stem from its ability to focus the illuminating light and the focal plane of the microscope objective on precisely the same point ( ). In most modern confocal microscopes a point light source is focused on to a small volume within the specimen and a confocal point detector is used to collect the resulting signal. This results in a reduction of the amount of out-of-focus signal from above and below the focal plane, producing a marked increase in both lateral ( x , y ) and axial ( z ) resolution ( ). Only one tiny area of the specimen is observed by each point source, so a useful full field of view must be gained by mechanically scanning the area of interest. By varying the plane of focus of both the source and detector within the tissue, the specimen can be optically ‘sectioned’ noninvasively, and detailed information on corneal structure determined ( ). Detailed descriptions of the optical principles involved in confocal microscopy can be obtained in various reviews ( ).
The microscope objectives most commonly used are nonapplanating water immersion objectives that are optically coupled to the cornea using a methylcellulose gel ( ). To obtain the maximum axial resolution (and hence optical sectioning) it is necessary to use a microscope objective with a large numerical aperture (which describes the light-gathering ability of the objective). However, such devices have a reduced field of view and shorter free working distances, which reduces the distance that the microscope can focus into the specimen from the surface ( ).
Tandem Scanning Confocal Microscopy
First-generation confocal microscopes used a modified Nipkow disc, which is a thin wafer with hundreds of pinholes that are arranged in a spiral pattern ( ). When a portion of the disc is placed in the internal light path of the confocal microscope, the spinning disc produces a scanning pattern of the subject. As the subject is inspected, light is reflected back through the microscope objective. The light that was reflected from in front of or behind the focal plane of the objective approaches the disc at an angle rather than perpendicularly. The pinholes of the disc permit only perpendicularly oriented rays of light to penetrate. This enables the microscope to view a very thin optical section of tissue. Because the illumination and detection of light through conjugate pinholes occur in tandem, this microscope was named the tandem scanning confocal microscope. The disadvantages of this system are a lack of a wide selection of objective lenses, an inability to control the signal-to-noise ratio directly at different tissue depths and the dramatic loss of light due to the fact that Nipkow discs transmit less than 1% of the available light ( ). This latter problem necessitates the use of a low-light-level camera to acquire the images, which limits frame speed acquisition.
Slit-Scanning Confocal Microscopy
The next-generation devices were variable-slit real-time scanning confocal microscopes ( ). In this design, two independently adjustable slits are located in conjugate planes. A rapidly oscillating two-sided mirror is used to scan the image of the slit over the plane of the cornea to produce optical sectioning in real time. This design has the advantages of optimal image contrast, enhanced clarity and decreased scan time, but it is more expensive than Nipkow-based systems, and z -axis quantification is not currently possible ( ).
Confocal Microscopy Through Focusing
Regardless of the technique used to obtain the images, the major problem associated with confocal microscopy relates to the interpretation and quantification of the data obtained. A technique called ‘confocal microscopy through focusing’ attempts to overcome this, by rapidly moving the focal plane of the objective lens through the entire cornea at a speed of approximately 80 µm/s, while x–y images are acquired at the focal plane. This means that approximately 450 sequential images (which are separated by approximately 1 µm) are acquired over the time taken to traverse the cornea (approximately 15 seconds). The cornea is then reconstructed using image-processing techniques, and an image is produced that is similar to a histological section, albeit in three dimensions in a living cornea ( Fig. 34.19 ).

Rostock Cornea Module
Heidelberg Engineering, in collaboration with Rostock University, Germany, has developed a novel digital confocal laser scanning microscope, a combination of Heidelberg retina tomograph (HRT) and the Rostock cornea module (RCM) ( ). The RCM uses the back-scattered light, similar to other confocal microscopes, with an interaction with the specimen producing a light signal that proceeds towards the detector. This device allows the operator to image sharply cellular structures and move through the different layers of the entire cornea, from epithelium to the endothelium. The RCM also enables the imaging of the peripheral areas of the cornea and conjunctiva. The instrument allows a scan depth of a maximum of 1500 µm, with an image size of 400 × 400 µm. The unit has an interchangeable 63 × standard microscopic lens, offers a choice of manual depth position adjustment and has an automatic brightness adjustment system. The CCD camera allows a permanent monitoring of the corneal contact on the screen. The RCM technology provides better image quality and produces a precise depth measurement compared with confocal slit-scanning microscopes ( ). Recently, a more compact version of the same instrument, the HRT III with RCM, has been introduced ( Fig. 34.20 ).

Using such techniques, confocal microscopy has provided valuable data on the structure and appearance of the cornea in many disease processes, including dystrophies, keratitis and endothelial disease. In addition, corneal changes following refractive surgery, corneal cross-linking, keratoplasty, in diabetics and due to contact lens wear have been documented ( ).
Measurement of Corneal Sensitivity
The cornea is richly innervated and is one of the most sensitive tissues in the body. Corneal sensitivity is a useful indicator of corneal disease and can help to determine physiological stress from wearing contact lenses ( ). Interest in assessing corneal sensitivity has increased over recent years, particularly in light of findings indicating that corneal sensitivity is significantly reduced in cases of dry eye and ocular surface disease ( ) and conjunctival sensitivity appears increased in symptomatic contact lens wearers and patients with reports of ocular dryness ( ).
Contact Aesthesiometry
Measurement of corneal sensitivity in the clinical setting has traditionally been achieved using a Cochet–Bonnet aesthesiometer ( Fig. 34.21 ). This device can be handheld or mounted on a slit lamp, and uses a single nylon thread to produce various forces, by varying its length in 0.5-cm steps (the longer the thread, the lighter is the force). The filament is lightly placed on to the cornea by the clinician using a support that allows manipulation in the x–y–z planes, while being viewed through the slit lamp. The subject reports when they can feel the thread touching the ocular surface, and the length of thread at which this occurs is recorded. The corneal touch threshold is defined as the length of the nylon filament at which the subject responds to 50% of the number of stimulations. This length is converted into pressure using a calibration curve and the reciprocal of this value gives the corneal sensitivity. Using this technique, it has been demonstrated that corneal sensitivity varies with surface location and is altered by age, iris colour, ambient temperature, time of day, contact lens wear and pregnancy ( ).

A number of factors complicate the use of such a device and can result in variations in the results obtained ( ). These include physical aversion to the approach of the device, problems with mounting the device accurately in the slit lamp and the impact of ambient humidity on the stiffness of the thread ( ).
Noncontact Aesthesiometry
Over the last 20 years a number of devices have been tested and developed to overcome the problems described above with contact aesthesiometry. All of these research instruments use noncontact means of stimulating the cornea. Initially, mechanical stimulation alone was investigated, but more recently aesthesiometers have been produced that stimulate the cornea using a variety of thermal, chemical or mechanical stimuli. These have included noncontact pneumatic devices which deliver compressed air as the stimulus ( ) and a device that measures both mechanical stimulation via an air puff and chemical stimulation via the administration of varying concentrations of carbon dioxide ( ).
With time, it is possible that devices based on these approaches will become commercially available, and aesthesiometry will become an important technique in contact lens practice.
Ocular Thermography
IR thermal imaging determines the wavelengths of IR radiation (or heat) emitted from a source and a specific region of interest. This technology was initially used in military settings for surveillance, and its application in other fields was restricted until the 1950s, when the technology was declassified ( ). Noncontact IR thermography has since been widely employed in a number of medical settings as a diagnostic tool ( ), including mass screening for fever in international airports during infectious disease epidemics ( ). The study of ocular surface temperature using IR thermography was first described in 1968 ( ) and IR thermography has since been used to study ocular physiology, including the relationship between ocular surface temperature and tear film measures, with notable interest in its application in dry eye disease ( ).
IR thermographers can vary in their features, however most are capable of working within temperature ranges between −20°C and 100°C, have a thermal resolution of 0.1°C, are sensitive to IR radiation between 8 and 14 µm, accurate within ±2%, exhibit frame speeds of up to 60 frames per second and frame sizes of 640 × 480 pixels ( ). Colour or monochromatic displays of the thermograms can be adjusted, according to user preference. Environmental conditions, such as ambient temperature, relative humidity and air flow can affect ocular surface temperature measurements and many clinical studies report controlling these variables, in addition to implementing a room adaptation period ( ). Keeping the alignment of the thermographer to measure the same region of interest between patients is important, as well as clearly defining the region of interest itself (e.g. central cornea, nasal or temporal conjunctiva and lids).
Most thermographers have been developed for measuring skin temperature, rather than ocular temperature and the Tomey TG-1000 Ocular Surface Thermographer (Tomey Corporation, Nagoya, Japan) is the only commercially available ocular-specific noncontact thermographer, but this has now been discontinued. The instrument was built using Tomey’s RC-5000 autorefractor/keratometer unit, with an additional IR camera module ( ). Several clinical studies have been conducted using this instrument ( ) and the device was able to detect differences in rates of cooling between patients with dry eyes and healthy controls ( ). Despite the current lack of commercially available ocular-specific thermographers, the continued interest in using thermography as a diagnostic tool ( ), particularly in the realm of ocular surface disease, means it is possible that ocular thermography may become more accessible in the future.
Assessment of Anterior-Segment Morphology
Morphology stems from Greek and relates to the ‘study of shape’. Over the past two decades, substantial improvements in computing technology has resulted in an explosion of the number of devices to determine the morphology of the AS, including the cornea, sclera, anterior chamber and lens. This section will concentrate on describing the devices available to determine various aspects of the shape of the cornea, limbus and sclera.
Devices that determine corneal shape are often referred to as ‘corneal tomographers’. Tomography stems from the Greek words for ‘slice’ (tomos) and ‘describing’ (graphia) ( ). AS tomography provides the topography of both the front and back corneal surfaces. A wide variety of methods exist, including those based on horizontal slit scanning ( ), rotational Scheimpflug scanning ( ) and OCT ( ). These devices can broadly be divided into those that determine only anterior corneal shape, those that can determine anterior and posterior corneal shape and finally technologies that can provide information on the cornea, limbus and sclera.
Anterior Corneal Shape
Keratometry
Knowledge of corneal curvature is primarily of interest as an aid in determining the initial contact lens to be placed on the eye in cases of rigid corneal lens fitting. In addition, an indication of rapid changes in curvature over time can be indicative of a compromised cornea and also aid in the diagnosis of keratoconus. Measurement of the radius of curvature of the cornea is based on the fact that the front surface of the cornea acts as a convex mirror. The reflection of an object (or mire, from the French for ‘target’) of known size at a known distance is viewed using a short-focus telescope, and a relatively simple equation allows the corneal front surface radius of curvature to be determined directly from the instrument. The corneal power that results from a given radius is often also indicated on the keratometer; alternatively, this can be calculated (see Appendix D ).
The optical principles of keratometry, the various types of keratometer available and their specific mode of operation may be obtained from other sources ( ). The actual region over which the standard keratometer measures corneal radius is that of two small areas approximately 1.5 mm on either side of the central fixation point ( ). Different types of keratometer use differently sized mires at differing separations. It is thus of no surprise that different keratometers may give differing radius values on the same eye.
Assessment of the curvature of excessively flat or steep (e.g. keratoconic) corneas with an optical keratometer may be difficult because the values may be beyond the range of many instruments. Conversion of a keratometer readings to its extended value can be determined by placing a +1.25 lens (for steep corneas) or a −1.00-D lens (for flat corneas) in front of the keratometer. Appendix E provides a table for determination of the extended value when such a procedure is adopted.
Most keratometers in clinical practice now use automated keratometry, using IR devices that rapidly and automatically determine central keratometry and refractive error simultaneously.
In addition to determining the central radius of curvature, it is useful to measure peripheral radius values, particularly in complicated conditions such as post penetrating keratoplasty and post refractive surgery. Conventional keratometers have traditionally been adapted by using peripheral fixation points ( ). However, in reality, keratometers cannot be used to determine corneal curvature accurately if the surface being measured does not have a constant radius of curvature or is not radially symmetrical ( ). For this reason, dedicated instruments using other technologies have been developed to measure overall corneal topography.
Corneal Topographic Analysis
The aim of corneal topography (or keratoscopy) is to describe accurately the shape of the corneal surface in all meridians ( ). In most cases, the technique uses a similar principle to keratometry, in that it determines the size of the image of a target reflected in the corneal surface, the primary difference relating to the fact that for keratoscopy a series of circular concentric targets are used (a Placido disc image). This arrangement allows both central and peripheral curvature to be determined. Historically, a photographic record of the corneal reflection images was made (photokeratoscopy) and measurements calculated subsequently ( ).
Modern-generation topographers capture the image electronically on a computer and use sophisticated image-processing software to provide immediate analysis of the reflected image (videokeratoscopy). Using this technique it has been clearly demonstrated that the cornea is aspheric and can best be described as a flattening ellipse, whose rate of flattening is asymmetrical about its centre ( ), with differences in anterior eye surface shape depending on refraction ( ). The history and detailed description of the development and operation of topographers and their clinical applications are described elsewhere ( ).
The simplest of topographers are reflective devices, which measure topography based on the reflection of mires from the anterior surface tear film, which are essentially identical in shape to the corneal surface.
Qualitative Assessment
The most basic reflective device for assessing corneal topography is the Placido disc, which is simply a series of concentric black and white rings on a flat circular disc with a central sight hole. The disc is positioned in front of the cornea and the reflections are observed. Using this method, only gross irregularities in the corneal surface and very high astigmatism can be detected. Improved versions of the Placido disc include the internally illuminated Klein keratoscope, Loveridge grid ( ), Tearscope-plus with corneal topography grid attachment ( ) and EASYTEAR View+ with placido grid insert ( ).
Quantitative Assessment
Quantitative reflective devices ( Fig. 34.22 ) utilize the same basic principle of projecting a grid on to the cornea. The images are captured with a video camera and a computational approach is adopted to analyse the data and derive a description for the corneal shape. The choice of computational method is important as this will largely dictate the accuracy and validity of the keratoscope. The most frequently utilized computational approach is the ‘slope of surface’ method ( ). Basically, devices that use this technology measure slope directly as a function of distance from a central reference axis and derive curvature from these results. It is important to note that these distance-based instruments are only estimating the average shape of the cornea, since the algorithms are based on a radially symmetrical surface, which does not accurately describe the cornea. These axial or sagittal measurements result in an underestimation of the radius of curvature in areas that may be steeper than the central cornea, and an overestimation in areas that are flatter. More recently, the algorithms have been modified and are now generally based on the radius of curvature, in an attempt to provide a better estimate of the local shape of the cornea.
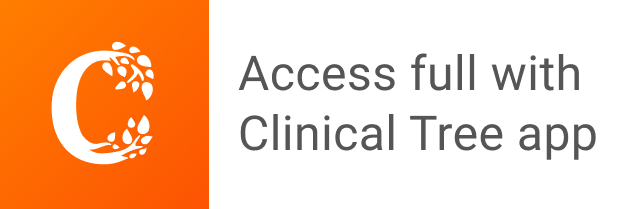