Introduction
A critical aspect of contact lens practice is monitoring the ocular response to lens wear, which ranges from acceptable physiological changes to adverse pathology. To do this, practitioners must possess a thorough understanding of the normal structure and function of the anterior eye, which is the subject of this chapter. In the course of reading other chapters in this book, the reader may need to refer back to this chapter on the functional anatomy and physiology of the anterior eye to develop a fuller understanding of the phenomena being described.
The Cornea
The cornea fulfils two important functions: together with the sclera, it forms a tough fibrous outer coat that encloses the ocular tissues and protects the internal components of the eye from injury. Significantly, the cornea also provides two-thirds of the refractive power of the eye. It is particularly well suited to its role: the cornea is curved and transparent, and the air–tear interface provides a refractive surface of good optical quality.
Corneal Anatomy
Gross Anatomy
The cornea is elliptical when viewed from in front, with its long axis in the horizontal meridian ( Table 2.1 ). This asymmetry is produced by a greater degree of overlap of the peripheral cornea by opaque limbal tissue in the vertical meridian. The surface area of the cornea is 1.1 cm 2 , which represents about 7% of the surface area of the globe ( ). Topographically, the cornea is conventionally divided into four zones (central, paracentral, peripheral and limbal). The central zone, which covers the entrance pupil of the eye, is spherical and approximately 4 mm wide and principally determines high-resolution image formation on the fovea. The paracentral zone, which lies outside the central zone, is flatter and becomes optically important in dim illumination when the pupil dilates. The peripheral zone is where the cornea is flattest and most aspheric ( ). Due to a difference in curvature between its posterior and anterior surfaces, the cornea shows a regional variation in thickness. Centrally the average thickness of the normal cornea based on a meta-analysis of studies in white adults is 535 µm (95% confidence interval, 474–596 µm) ( ), with a peripheral thickness between 11% and 19% higher than in the centre ( ).
Parameter | Value |
---|---|
Area | 1.1 cm 2 |
Diameter | |
Horizontal | 11.8 mm |
Vertical | 10.6 mm |
Radius of curvature | |
Anterior central | 7.8 mm |
Posterior central | 6.5 mm |
Thickness | |
Central | 0.54 mm |
Peripheral | 0.67 mm |
Refractive index | 1.376 |
Power | 42 D |
Microscopic Anatomy
When the cornea is viewed in the transverse section, five distinct layers can be resolved: epithelium, anterior limiting lamina (Bowman’s layer/membrane), stroma, posterior limiting lamina (Descemet’s membrane) and endothelium ( Fig. 2.1 ).

Epithelium
The epithelium represents approximately 10% of the thickness of the cornea (55 μm) ( ). It is a stratified squamous nonkeratinized epithelium, consisting of five to six layers of cells ( Fig. 2.2 ), which undergo a constant process of cyclic shedding and replacement to maintain corneal integrity. Three distinct epithelial cell types are recognized: a single row of basal cells, two to three rows of wing cells and two to three layers of superficial (squamous) cells. In addition, several nonepithelial cells are present (e.g. lymphocytes, macrophages and dendritic cells). The epithelium forms a permeability barrier to water, ions and hydrophilic molecules above a certain size, as well as forming an effective barrier to the entry of pathogens. Further epithelial specialization enhances adhesion between cells, to withstand shearing and abrasive forces. Furthermore, throughout the thickness of the epithelium, adjacent cells are connected to one another by water channels (aquaporins) that are engaged in transcellular water transport and gap junctions to allow the transfer of ions and small molecules between cells ( ).

Superficial cells are structurally modified for their barrier function and interaction with the tear film. Scanning electron microscopy of surface cells shows extensive finger-like and ridge-like projections (microvilli and microplicae), which increase the epithelial surface area. Light, medium and dark cells can be distinguished depending on the number and pattern of surface projections ( ). It has been suggested that dark cells, which are relatively free of these surface features, are close to being desquamated into the tear film. By contrast, the newly arrived light cells possess a more extensive array of surface projections. In high-power transmission electron micrographs, microvilli and microplicae show an extensive filamentous covering known as the glycocalyx. The glycocalyx is formed from membrane-bound mucin glycoproteins and is important for spreading and attachment of the precorneal tear film. In accordance with their barrier function, a complex network of tight junctions links superficial cells that exclude water-soluble dyes such as fluorescein ( ). However, loss of the integrity of the epithelium as a result of a variety of contact lens-related factors including: trauma, desiccation, infection and solution-induced toxicity can lead to a variety of fluorescein staining patterns ( ).
Wing cells are so named because of their characteristic shape, with lateral extensions and a concave inferior surface to accommodate the apices of the basal cells. Their nuclei tend to be spherical or elongated in the plane of the cornea. The cell borders of the polygonal wing cells show prominent infoldings that interdigitate with adjacent cells, and numerous desmosomes. This arrangement results in strong intercellular adhesion. The cytoplasm contains prominent cytoskeletal elements (predominantly actin and cytokeratin intermediate filaments), and although the usual complement of organelles is present, they are few in number.
Basal cells consist of single-layer columnar cells with a vertically oriented oval nucleus. Ultrastructurally, they are similar in appearance to wing cells. The plasma membrane similarly shows pronounced infolding, and the cytoplasm contains prominent intermediate filaments. A variety of cell junctions are present, including desmosomes, which mediate adhesion between cells; hemidesmosomes, which are involved in the attachment of basal cells to the underlying stroma; and gap junctions, which allow for intercellular metabolic coupling. Basal cells form the germative layer of the cornea, and mitotic cells are often seen at this level.
Basal Lamina and Bowman’s Layer
The basal lamina (basement membrane) is synthesized by basal cells. It varies in thickness between 0.5 and 1 μm and under the electron microscope can be differentiated into an anterior clear zone (lamina lucida) and a posterior darker zone (lamina densa). The basal lamina is part of a complex adhesion system, which mediates the attachment of the epithelium to the underlying stroma ( Fig. 2.3 ). Hemidesmosomes link the cytoskeleton via a series of anchoring fibrils to anchoring plaques in the anterior stroma. The molecular components of this adhesion complex have been identified and include type VII collagen, integrins, laminin and bullous pemphigoid antigen ( ).

Bowman’s layer (anterior limiting lamina) varies in thickness between 8 and 14 μm. With the light microscope, it appears as an acellular homogeneous zone. Ultrastructurally, it is composed of a randomly oriented array of fine collagen fibrils, which merge with the fibrils of the anterior stroma ( ). Fibrils are composed primarily of collagen types I, III and V. Collagen VII, associated with anchoring fibrils, is also present. There is evidence that Bowman’s layer is formed and maintained primarily by the epithelium, although its function is unclear. The absence of Bowman’s layer from the cornea of most mammals and the fact that corneas are devoid of this layer over the central cornea following photorefractive keratectomy apparently function normally suggest that it is not critical to corneal integrity ( ).
Stroma
The stroma is approximately 500μm thick and accounts for 90% of the thickness of the cornea. It is composed predominantly of collagen fibrils (70% dry weight) embedded in a highly hydrated matrix of proteoglycans ( ). A variety of collagen types have been identified. Type I is the major fibril-forming collagen, with lesser amounts of types III and V. Nonfibril-forming collagens, including types VI and XII, are found in the interfibrillar matrix ( ). A section taken perpendicular to the corneal surface reveals that the collagen fibrils are arranged in 200–250 layers (lamellae) running parallel to the surface ( Fig. 2.4 ). Lamellae are approximately 2 μm thick and 9–260 μm wide and extend from limbus to limbus. Fibrils of adjacent lamellae make large angles with each other. In the superficial stroma, the packing density and tangles between lamellar are less than 90 degrees, but lamellae become less densely packed and orthogonal in the deeper stroma ( ; ). This preferred orthogonal orientation gradually changes in favour of circumferentially aligned collagen at the limbus. This particular arrangement of collagen imparts a high tensile strength for corneal protection, which is important given its exposed position. Within lamellae, all collagen fibrils are parallel with uniform size and separation ( Fig. 2.5 ). Accurate physiological measurements of collagen fibre diameter and spacing can be obtained for the hydrated cornea with the aid of X-ray diffraction. Using this technique, the mean fibril diameter in the human cornea is 31 nm, with an interfibrillar spacing of 55 nm ( ). This narrow fibril diameter and constant separation, which is a characteristic of corneal collagen, are necessary prerequisites for transparency.


The interfibrillar space contains a matrix of proteoglycans (approximately 10% of dry weight). These molecules are highly sulphated, and along with bound chloride ions create a polyanionic stromal interfibrillar matrix that induces osmotic swelling. As well as playing a major role in corneal hydration, collagen–proteoglycan interactions are also thought to be important in determining the size and spatial arrangement of stromal collagen fibrils ( ; ).
Collagen and proteoglycans are maintained by keratocytes. These cells occupy 3%–5% of stromal volume and lie between collagen lamellae, flattened in the plane of the cornea ( Fig. 2.6 ). Keratocyte density examined by confocal microscopy and biochemical methods ( ; ) is nonuniform. Density decreases from superficial to deep stroma ( ) and increases from centre to periphery. Keratocytes display a large central nucleus and long slender processes extend from the cell body. Processes from adjacent cells sometimes make tight junctions with each other. Cell organelles are not numerous but the usual complement of organelles, including endoplasmic reticulum, Golgi apparatus and mitochondria, can be observed ( ).

Newer lamellar corneal transplantation techniques have been developed that allow selective replacement of the diseased corneal layers. Deep anterior lamellar keratoplasty (DALK), which is increasingly being used to treat keratoconus and corneal scarring, involves the replacement of the affected stroma whilst retaining the host’s healthy Descemet’s membrane and endothelium.
Lamellar corneal transplant techniques such as DALK and Descemet’s stripping endothelial keratoplasty (DMEK), are becoming increasingly popular. During these procedures, air or fluid is injected into the donor cornea to separate the endothelium and Descemet’s membrane. performed a histological examination of donor corneas using air bubble separation of the posterior stroma and controversially claimed to have identified a novel pre-Descemet’s posterior stromal layer, termed ‘Dua’s layer’, which was widely publicized. However, the current consensus amongst corneal experts is that this layer is not sufficiently unique to constitute a new corneal layer ( : ).
Descemet’s Membrane
Descemet’s membrane (posterior limiting lamina) is the basement membrane of the corneal endothelium. It lies between the endothelium and the overlying stroma ( Fig. 2.7 ). At birth, it is 3–4 μm thick and increases to a thickness of 10–12 μm in the adult. In the periphery of aged corneas, Descemet’s membrane displays periodic sections of thickening, which are known as Hassall–Henle warts. The anterior one-third of Descemet’s membrane represents that part produced in foetal life and, under the electron microscope, is characterized by a periodic banded pattern ( Fig. 2.8 ). The posterior two-thirds, which is formed postnatally, has a more homogeneous granular appearance. Descemet’s membrane has a unique biochemical composition in contrast with other basement membranes ( ). The major basement membrane collagen type is type IV, whereas in Descemet’s membrane-type VIII collagen predominates.


Endothelium
The endothelium is a monolayer of squamous cells that lines the posterior surface of the cornea ( Fig. 2.9 ) and plays a critical role in maintaining corneal transparency ( ). As it has a limited capacity for mitosis to replace damaged or effete cells, there is a progressive reduction in endothelial cell number with age. At birth, the cornea contains a total of approximately 500,000 cells, which represents a mean density of 4500 cells/mm 2 . During infancy, cell loss is particularly marked and a 26% reduction occurs in the first year of life ( ). Thereafter, the rate of loss progressively declines into old age. Since grafted corneas appear to maintain transparency and functional normality with an endothelial cell density of less than 1000 cells/mm 2 , it seems that normal cell density represents a considerable ‘physiological reserve’ ( ). When viewed en face, for example using a specular microscope, the endothelium appears as a mosaic of polygonal (typically hexagonal) cells ( ). In response to pathology, trauma, age and prolonged contact lens wear, the endothelial mosaic becomes less regular and shows a greater variation in cell size (polymegethism) and shape (pleomorphism) as cells spread to fill gaps caused by cell loss. Under the electron microscope, the lateral borders of the cells are markedly convoluted and adjacent cells are linked by tight junctions (with less-frequent gap junctions) ( ). The complement of organelles seen in endothelial cells reflects their high metabolic activity, with numerous mitochondria and a prominent rough endoplasmic reticulum.

Corneal Innervation
Source and Distribution of Corneal Nerves
The cornea is the most richly innervated surface tissue in the body. Corneal nerves are responsible for the detection of somatosensory stimuli and play an important role in initiating the blink reflex, wound healing and tear secretion (see , for a recent review). The majority of corneal nerves are sensory and derive from the nasociliary branch of the trigeminal nerve ( ). There is also evidence for the existence of a modest sympathetic innervation from the superior cervical ganglion ( ). Branches from the nasociliary nerve either pass directly to the eye as long ciliary nerves or traverse the ciliary ganglion, leaving it as short ciliary nerves that enter the eye close to the optic nerve. Nerves destined for the cornea travel initially in the suprachoroidal space, before crossing the sclera to advance radially towards the cornea.
Most of the 50–80 precorneal nerve trunks, which contain a mixture of myelinated and unmyelinated fibre bundles, enter the cornea at the mid-stromal level. Myelin is soon lost and the unmyelinated nerve fibre bundles divide repeatedly and move anteriorly to form a rich plexiform network in the anterior one-third of the stroma. Axons are particularly dense immediately beneath Bowman’s layer, forming an extensive subepithelial plexus ( ). From this plexus, axons pass vertically through Bowman’s layer, losing their Schwann cell sheath in the process. Upon entering the epithelium, axons turn through 90 degrees and divide into a series of fine branches that course between basal cells ( Fig. 2.10 ). Some branches pass into the more superficial layers before terminating. The density of nerve terminals is greatest centrally, corresponding to approximately 600 terminals/mm 2 , which results in large overlapping receptive fields ( ).

Corneal nerves display complex neurochemistry. A variety of neurotransmitters and neuromodulators have been identified, including acetylcholine, substance P and calcitonin gene-related peptide. However, it is unclear how these particular neurochemicals correlate with function ( ).
Functional Considerations
Corneal nerves serve important sensory, reflex and trophic functions. Interest in the sensitivity of the cornea dates back to the 19th century ( ) when the pioneering German physiologist von Frey concluded that pain was the only sensation perceived by the cornea. This was consistent with his theory of the specificity of sensory receptors, which maintained that each sensory modality was subserved by a separate anatomically distinct nerve terminal. In his experiments on the cornea, von Frey could elicit only a sensation of pain and, as the cornea contained exclusively free (unspecialized) nerve endings, he concluded that free nerve endings were the exclusive receptors for pain. Although the specificity theory has subsequently been challenged, particularly with respect to its exclusivity, the question as to whether pain is the only sensory modality perceived by the cornea remains.
Modern experiments using carefully controlled corneal stimulation, with a variety of mechanical, chemical and thermal stimuli, have evoked only sensations of irritation or pain. By contrast, electrophysiological studies of corneal afferent neurones have identified neurones that respond to mechanical, thermal and chemical stimulation. However, since the conscious perception of these sensations has not been demonstrated, it is likely that such specificity of modality is lost during central nervous system processing. The electrophysiological recording also allows for the mapping of receptive fields. These are often large and overlapping, which explains the inability of the cornea to localize a stimulus accurately ( ). The sensitivity of the cornea to mechanical stimulation is particularly acute and acts as a trigger for the protective blink and lacrimal reflexes. Cold receptors may be important in signalling evaporative cooling, which is a major determinant of spontaneous eye-blink frequency ( ).
Corneal afferent fibres also exert important trophic influences and are essential for maintaining ocular surface integrity. Damage to corneal sensory nerves by surgery, trauma or infection produces neuroparalytic keratitis – a condition that is characterized by progressive epithelial cell loss and oedema. The mechanism of this trophic role is not fully understood, although the release of neuropeptides (e.g. substance P and calcitonin gene-related peptide) may be a factor. Sympathetic nerves also play a role in epithelial maintenance by regulating ion transport processes, and cell proliferation and migration during wound healing.
Corneal Metabolism
Source of Oxygen and Nutrients
To perform its vital functions, the cornea requires a constant supply of oxygen and other essential metabolites (e.g. glucose, vitamins and amino acids). However, its avascularity dictates that alternative routes must exist for the provision of its metabolic needs. There are three possibilities: from the perilimbal vasculature, from the tear film or from the aqueous humour. In open-eye conditions, the bulk of the oxygen required for the cornea is obtained from the atmosphere via diffusion across the precorneal tear film. Under steady-state conditions, it can be assumed that the tears are saturated with oxygen, and therefore at an oxygen tension corresponding to the atmosphere (155 mmHg at sea level). It has been estimated that the oxygen tension of the aqueous humour in the human eye lies between 30 and 40 mmHg ( ).
Experiments using nitrogen-filled goggles or sealed scleral lenses have shown the corneal dependence on tear-side oxygen to avoid oedema and maintain normal function. The reason why the cornea swells during contact lens wear is explained in Fig. 2.11 . During eye closure, the oxygen level in the tears is in equilibrium with the palpebral vasculature (55 mmHg) ( ).

Significantly, the corneal thickness increases by approximately 5% during sleep and returns to baseline levels within 1 hour of eye opening. It has been suggested that overnight swelling is related to tear film tonicity rather than reduced oxygen availability ( ). The oxygen flux into the cornea can be measured using polarographic oxygen sensors. It is in the region of 6 μL/cm 2 /h for the cornea as a whole, although the consumption rate for its composite layers is not equal. Consumption rates have been estimated as 40:39:21 for the epithelium, stroma and endothelium, respectively ( ).
Several lines of evidence indicate that the aqueous humour is the primary source of glucose and essential amino acids for the cornea ( ). The glucose concentration of tears is low compared with that in the aqueous humour, and the insertion of nutrient-impermeable implants into the stroma results in degeneration of the tissue lying anterior to the implant. Although exogenous glucose is primarily utilized, glycogen stores are present in all corneal cells to provide glucose in conditions of metabolic stress.
The role of the perilimbal vasculature in the provision of oxygen and nutrients appears limited and it is likely that it is significant only for the corneal periphery ( ).
Oxidative Metabolism
The cornea derives its energy principally from the oxidative breakdown of carbohydrates ( ). Glucose, which is the primary substrate for the generation of adenosine triphosphate (ATP), is catabolized by three metabolic pathways: glycolysis, the tricarboxylic acid (TCA) (Krebs) cycle and the hexose monophosphate shunt ( Fig. 2.12 ). Anaerobic glycolysis accounts for the majority (85%) of glucose metabolism. In this pathway, glucose is first oxidized to pyruvate and then subsequently reduced to lactate, with a net yield of two molecules of ATP per mole of glucose. The TCA cycle results in a greater energy yield (36 ATP). This pathway is most active in the corneal endothelium, which has the greatest energy requirement.

Metabolic waste products can be potentially damaging if allowed to accumulate. Although carbon dioxide can readily diffuse out of the cornea across its limiting layers, lactate is less easily eliminated. Under normoxic conditions, lactate is able to diffuse slowly across the endothelium into the anterior chamber. However, during periods of hypoxia the proportion of glucose that is metabolized anaerobically increases. The resulting accumulation of lactate causes stromal oedema via an increased osmotic load ( ) and localized tissue acidosis ( ).
The hexose monophosphate shunt (also known as the pentose phosphate shunt) plays an important role in the corneal epithelium ( ), where it fulfils several important functions, including the generation of intermediates for biosynthetic reactions and the prevention of oxidative damage by free radicals.
Corneal Transparency
Under normal conditions the cornea is highly transparent, transmitting more than 90% of incident light. Structurally, the cornea is a typical connective tissue consisting principally of a matrix of collagen and proteoglycans. Under normal circumstances, such an arrangement would favour light scatter with consequent loss of transparency. This raises two fundamental questions: how is transparency achieved and how is it maintained? To begin to answer these questions, it is necessary to understand the spatial organization of the stromal matrix and the importance of corneal hydration control.
Stromal Organization
explained the transparency of the cornea on the basis of the uniform diameter and regular separation of the stromal collagen. He suggested that the collagen fibrils of the stroma were disposed in a regular crystalline lattice and that light scattered by the fibrils is eliminated by destructive interference in all directions other than the forward direction. This situation will hold as long as the axes of the collagen fibrils are arranged in a regular lattice with a separation less than the wavelength of light. It has been suggested, however, that the fibrillar arrangement need not be in a perfect crystal lattice to maintain transparency ( ), although disruption of short-range order between fibrils will lead to increased scatter and a loss of transparency.
The factors involved in the maintenance of collagen fibril size and spatial order are not fully understood. It has been proposed that collagen fibril diameters may be controlled by the incorporation of minor collagens (e.g. type V) into the predominantly type I fibrils ( ) and that their spatial separation is a function of proteoglycan–collagen interactions ( ). Proteoglycans are a family of glycoproteins that consist of a protein core to which are attached sugar chains of repeating disaccharide units termed glycosaminoglycans (GAGs). These molecules are increasingly being recognized as important prerequisites for transparency ( ; ). Proteoglycans were originally classified according to their GAG composition; however, current nomenclature groups them into families based on homologous sequences of amino acids in their protein core. Corneal stromal proteoglycans are members of the small leucine-rich family, which are small enough to fit in the space between collagen fibrils. The most prevalent GAG in the cornea is keratan sulphate, which is found in three types of proteoglycan: lumican, keratocan and mimecan ( ; ). The other type of corneal proteoglycan is decorin, which contains a hybrid chondroitin sulphate/dermatan sulphate side chain. Evidence from several sources has shown that lumican and decorin play important roles in regulating collagen fibril diameter and maintaining the spacing between fibrils once formed, which are essential for transparency.
Hydration Control
The state of corneal hydration is another important determinant of corneal transparency ( ). The hydrophilic properties of the stroma are to a large part determined by proteoglycans, which contribute to the fixed negative charge of the stroma and produce a passive gel swelling pressure through electrostatic repulsion ( ). Physiologically, corneal hydration is maintained at approximately 78%. If the cornea is allowed to swell ±5% of this value, it begins to scatter significant quantities of light ( ).
Maintenance of physiological corneal hydration is to a large part dependent on the corneal endothelium, which possesses both a barrier property and a metabolically driven pump. The endothelial barrier to the free passage of molecules from the aqueous humour is formed principally by focal tight junctions between adjacent endothelial cells. However, in contrast to other barrier epithelia, these junctions are of low electrical resistance and allow the passage of ions and small molecules. This leak is offset by the metabolically driven pumping of ions out of the stroma by the endothelium, which maintains a transcellular potential difference (aqueous side negative) to balance stromal swelling pressure ( ). Disruption of this osmotic gradient will result in stromal fluid imbibition.
The specific endothelial ion transport mechanisms responsible for the maintenance of physiological hydration are not fully understood. A simplified model representing our current level of knowledge is represented in Fig. 2.13 . There is compelling evidence that a flux of bicarbonate ions is the predominant component of the endothelial ion transport system ( ). Subsequent studies have identified that Cl − transporters may also be important in maintaining the pump ( ). The bicarbonate is generated either by a Na + /HCO 3 − co-transporter located on the basolateral plasma membrane or via the intercellular conversion of carbon dioxide by the enzyme carbonic anhydrase. Bicarbonate leaves the cell via an apical bicarbonate ion channel. The driving force for the bicarbonate flux is generated by a sodium–potassium ATPase that resides on the basolateral endothelial membrane. The energy associated with subsequent sodium re-entry (via Na + /H + and Na + /HCO 3 − transporters) is coupled to active HCO 3 − flux ( ).

The epithelium also contributes to corneal hydration control ( ). The tight junctions between superficial epithelial cells form an effective permeability barrier to ions and polar solutes. For example, the anionic molecule sodium fluorescein does not penetrate an intact epithelium. However, damage to the superficial cells allows fluorescein to enter the epithelium, with resulting corneal staining. In addition to its barrier properties, the epithelium also possesses active ion transport systems for Na + and Cl − . As these pumps contribute to the tonicity of the tear film, it is likely that they are involved in the maintenance of stromal hydration.
Response to Oedema
When corneas swell, light scattering increases, with an ensued transparency loss due to the disruption of the regular collagen matrix. The collagen fibrils themselves swell very little and most of the additional water goes into the interfibrillar spaces. Transmission electron micrographs of oedematous corneas show fibril aggregation, with the result that large areas are devoid of collagen fibrils ( ). There is evidence that collagen aggregation occurs as a result of loss of GAGs, which previously had maintained fibre separation ( ).
Corneal Epithelial Wound Healing
A smooth and intact corneal epithelium is necessary in order for the cornea to maintain clear vision. The corneal epithelium has a very high turnover rate, and under homoeostatic conditions, it is regenerated every 5–14 days. Due to its exposed position, the cornea is potentially vulnerable to a variety of external insults. It possesses several protective mechanisms to avoid injury, but if tissue damage occurs it is capable of an effective wound-healing response ( ; ). Corneal epithelial repair is a complex process involving an orchestrated interaction between cells and the extracellular matrix, which is coordinated by a variety of growth factors. The process can be divided into three phases: (1) initial covering of the denuded area by cell migration, (2) cell proliferation to replace lost cells and (3) epithelial differentiation to re-form the normal stratified epithelial architecture.
Following a full-thickness epithelial defect, fibronectin, an adhesive glycoprotein, is synthesized and covers the surface of the bared stroma where it serves as a temporary matrix for cell migration. The adhesion between fibronectin and the epithelium is mediated by integrin–matrix interactions (integrins are a family of cell surface receptors that bind to certain extracellular matrix proteins). Several growth factors have been implicated in the control of the wound-healing response, including epidermal growth factor, transforming growth factor-beta, platelet-derived growth factor and fibroblast growth factor ( ). Growth factors, which are produced by a variety of sources (e.g. ocular surface epithelia and the lacrimal gland), are able to regulate the process of epithelial migration, proliferation and differentiation. There is evidence that epithelial–stromal interactions play an important role in corneal wound healing ( ). Epithelial injury triggers keratocyte apoptosis (programmed cell death) in the anterior stroma, via the release of apoptosis-inducing cytokines from epithelial cells. Keratocyte apoptosis subsequently triggers a wound-healing cascade, which influences epithelial repair.
Regeneration of the corneal epithelium is highly dependent on the integrity of the limbus ( ; ). Cumulative evidence indicates that a proportion of limbal basal epithelial cells possess the properties of stem cells, which are ultimately responsible for corneal epithelial replacement. Stem cells have several unique characteristics: they are poorly differentiated and long lived and have a high capacity for self-renewal. When these cells divide, one of the daughter cells replenishes the stem cell pool, whilst the other is destined to undergo further cell divisions before differentiating. Such a cell is referred to as a transient amplifying cell. Transient amplifying cells undergo several rounds of cell division before fully differentiating. These cells play an important role in epithelial wound healing, where their proliferative capacity is increased by shortening cycle times and increasing the number of times that the transient amplifying cells can divide before maturation.
The Ocular Adnexa
The ocular adnexa is those structures that support and protect the eye and include the eyelids, conjunctiva and lacrimal system. They play an important role in the formation of the preocular tear film and collectively defend the eye against antigenic challenges.
Eyelids
The eyelids are two mobile folds of skin that perform several important functions: they act as occluders that shield the eyes from excessive light, and through their reflex closure, they afford protection against injury. The lids also form a precorneal tear film of uniform thickness during the upturn phase of each blink. The action of blinking is also important for tear drainage.
Gross Anatomy
The eyelids are joined at their extremities, termed ‘the canthi’, and when the eye is open, an elliptical space, the palpebral fissure, is formed between the lid margins. In a normal adult, the vertical width of the palpebral fissure ranges from 8 to 11 mm and its horizontal length from 27 to 30 mm ( ).
In the primary position, the upper lid, which is the larger and more mobile of the two, typically covers approximately the upper third of the cornea, whilst the lower lid is level with the inferior corneal limbus ( Fig. 2.14 ). Important differences in eyelid anatomy exist between Asian and Caucasian eyes. A prominent feature of Asian eyes is an absent or very low eyelid skin fold, which together with an inferior extension of the preaponeurotic fat pad gives rise to a fuller upper eyelid appearance and a narrower palpebral fissure ( ). Furthermore, Asians typically have a skin flap, the epicanthus, which begins laterally in the superior palpebral fold and progresses medially to cover the medial canthus

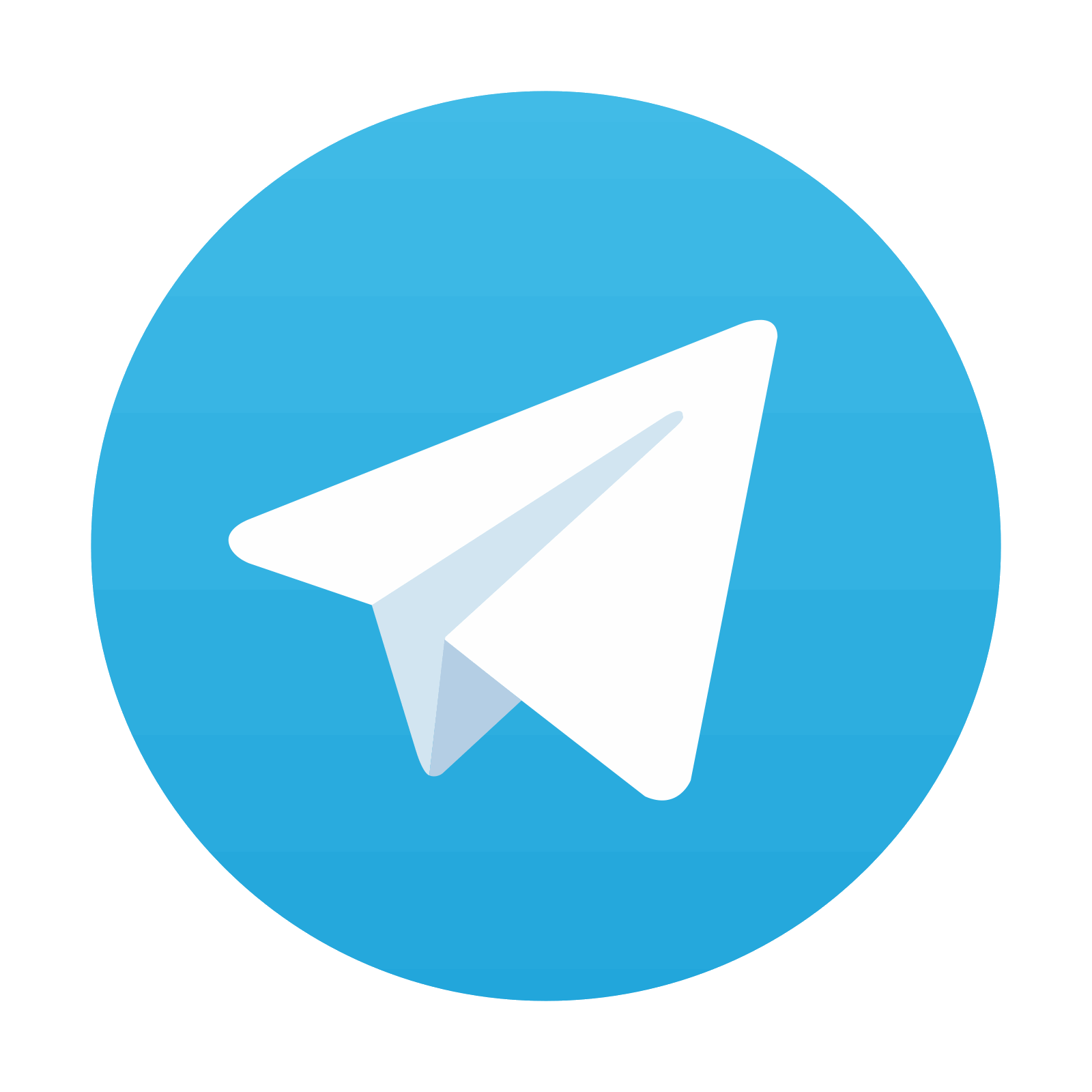
Stay updated, free articles. Join our Telegram channel
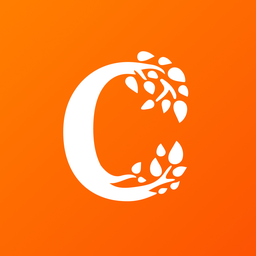
Full access? Get Clinical Tree
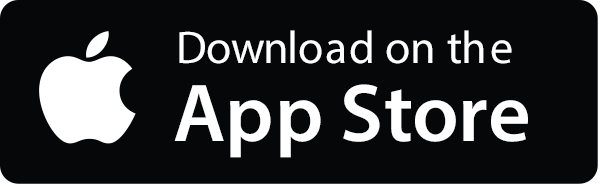
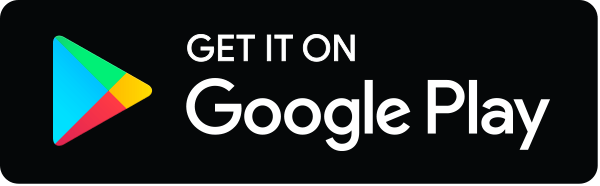
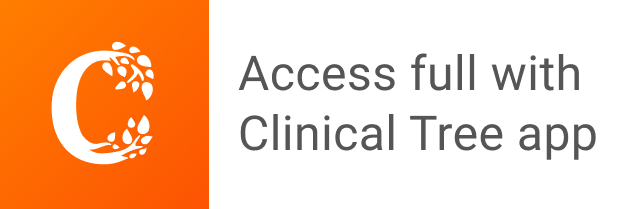