Development of the Visual System
T. Rowan Candy
Vision forms one of the sensory inputs that allow us to interact with our environment. For visual information to be integrated with information from our other senses, a representation of the visual environment needs to be created in a language that the brain can interpret. This is a language of electrical potentials. The chief role of the first stages of the visual system, therefore, is to convert an image of the world into interpretable electrical potentials.
The adult eye and visual system are able to achieve this goal. An image of the external world is formed on the retina and information contained in this image is converted into membrane voltages in approximately 120 million photoreceptors. The information from these photoreceptors is then transmitted in an orderly fashion into the visual cortex and beyond. To ensure that patients can enjoy this facility, the challenge for vision scientists and clinicians is to understand how a fully healthy system forms, how an abnormal system can arise, and how to treat or prevent abnormality.
The goal of this chapter is to provide our understanding of the development of the structures of the visual system. The discussion is split into two sections that represent the two major challenges: to build a transparent optical system capable of forming a continually focused image of the external world and to build a neural system that can transmit information reliably yet recalibrate constantly as a child grows. Recent research has shown that these systems are largely constructed according to a map and timetable defined by the transient expression of genes (1,2), with modulation and refinement guided by the local environment and ultimately neural activity (3,4).
Building A Transparent Optical System
The optical components of the adult eye consist of the cornea, pupil, and lens to a first approximation. The aqueous and vitreous humors inflate the eye to maintain these components at the appropriate distances from the retinal image plane and support the needs of the structures that must remain transparent without clouding from blood vessels. The following section describes how this ultimately transparent and aligned optical system forms in humans. Most of this information has been gathered through light and electron microscopy studies of human tissue, which have been extensively reviewed in books (5,6,7). These reviews form the basis of the following section, together with information from Ozanic and Jakobiec (8).
The Embryonic Period (First 2 Months of Gestation)
The first 8 weeks of gestation forms the embryonic period during which fundamental ocular structures are forming. Abnormal events during this period (e.g., infection or trauma) will result in relatively major structural malformations of the eye and visual system.
The first evidence of the eyes appears in the human embryo at approximately 22 days of gestation, just 3 weeks after conception (9). The neural ectoderm, which goes on to form the nervous system, is rolling up to form a tube along the length of the embryo. At this point, the embryo is still less than 1 cm long. One end of this developing neural tube is destined to become the brain and already consists of three sections, the prosencephalon (forebrain), mesencephalon (midbrain), and rhombencephalon (hindbrain). The first evidence of the eyes appears in the internal wall of the rolling tube in the mesencephalon (between the soon-to-form telencephalon and diencephalon) (Fig. 1.1A). The evidence consists of a small section of the wall on each side of the head starting to bulge out toward the surface of the embryo. These evaginations (optic primordia, or sulci) continue to extend while the rest of the neural tube seals—the tube is complete at approximately 24 days. The evaginations extend to form a vesicle and stalk whose hollow center is continuous with the brain (Fig. 1.1B, Fig 1.2A). This optic vesicle forms parts of the eye, and the optic stalk forms the path for the later neural connections from the eye to the brain (Fig. 1.1C, Fig 1.2B).
The external layer of the embryo consists of surface ectoderm. As the optic stalk and vesicle extend, the proximity of the optic vesicle to the surface ectoderm causes the nearest section of surface ectoderm to thicken and start on a path to becoming the lens of the eye. This area of thickening forms on day 27 (8) and is called the lens placode (Fig. 1.1B). The fact that the surface ectoderm will only form the placode if the vesicle is present demonstrates the importance of local interactions between cells in normal development (10). The optic vesicle is said to induce the lens placode. The following day, the presence of the surface ectoderm starts to induce the closest section of the optic vesicle to form a thickened retinal disk (Fig. 1.1B). Abnormalities at this early point in gestation lead to relatively severe malformations such as anophthalmia or microophthalmia (see Chapters 12 and 13 on anterior and posterior chamber abnormalities).
Formation of the Optic Cup and Basic Optical Structures
The next step, at the end of the fourth week, is for the optic vesicle and lens placode to each fold back on themselves and invaginate toward the brain. The folding of the optic vesicle, which
starts a couple of days before that of the surface ectoderm, creates the formation of a two-layered cup—the optic cup—and the folding of the surface ectoderm creates the lens vesicle. This is a hollow ball of cells that will completely pinch off from the surface ectoderm at 33 days and lie in the mouth of the optic cup (Fig. 1.1C, Fig. 1.2B). This mouth will become the future pupil.
starts a couple of days before that of the surface ectoderm, creates the formation of a two-layered cup—the optic cup—and the folding of the surface ectoderm creates the lens vesicle. This is a hollow ball of cells that will completely pinch off from the surface ectoderm at 33 days and lie in the mouth of the optic cup (Fig. 1.1C, Fig. 1.2B). This mouth will become the future pupil.
![]() Figure 1.2. Tissue corresponding to the schematic diagrams in Figure 1.1A. A: A section through the forebrain of a 4-week-old human embryo showing the closed neural tube, optic vesicles, and future optic stalks (corresponding to Figure 1.1B before the formation of the lens placode and retinal disk). (From Hamilton WJ, Boyd JD, and Mossman HW. Human Embryology: prenatal development of form and function, 4th ed. Philadelphia: JB Lippincott; 1972 .) B: A 5-week-old human embryo with optic cup and lens vesicle (corresponding to Figure 1.1C). (FromSmelser GK. Embryology and morphology of the lens. Invest Ophthalmol 1965;44:398–410 , with permission.) |
The other main optical component of the eye, the cornea, is formed from the surface ectoderm that sits over the lens vesicle and mouth of the optic cup after they have invaginated. Once the lens vesicle has disconnected, this tissue invaginates again to form the surface of the future eyelids and parts of the cornea (the continuous conjunctival sac) (Figs. 1.3 and 1.4B). The future margins of the eyelids start growing toward each other, finally meeting and fusing along the border. The corneal section of surface ectoderm forms the future corneal epithelium, which initially only consists of two layers of cells resting on a basement membrane. The size of the future cornea has been shown in an avian model to depend on the size of the optic cup. A small cup implies a small but normal cornea (11)—another indication of local interactions.
Thus, in the fifth week of gestation, the future lens is already sitting behind the primitive cornea, in the mouth of the optic cup, and is
positioned to form an image on the developing retina. The basal lamina of the surface ectoderm lies on the outside of the lens vesicle and later thickens to form the capsule around the mature lens.
positioned to form an image on the developing retina. The basal lamina of the surface ectoderm lies on the outside of the lens vesicle and later thickens to form the capsule around the mature lens.
During the fifth to seventh weeks, the hollow vesicle of lens cells fills as it starts to take its mature form (induced by the proximity of the optic cup). The cells in the posterior wall, closest to the retina, start to elongate forward to fill in the hollow volume (Figs. 1.4 and 1.5). They continue forward until they meet the anterior cells at 45 days and the lens becomes solid. This is the embryonic lens nucleus. The nucleus is approximately spherical and the fibers within it are named the primary lens fibers. The optic cup then induces the cells from the anterior surface of the lens to migrate around to the vertical equator. The organelles in these cells begin to disintegrate and the cells elongate to form more fibers. The fiber tips extend over the primary lens fibers beneath the lens capsule, forward to the anterior pole and backward to the posterior pole of the lens. The tips of the fibers extending from cells at the different locations around the equator all meet to form the clinically visible anterior and posterior sutures.
The first layer of secondary fibers is already in place in the seventh week of gestation and the further fibers are laid down sequentially in layers. Thus, depending on the timing of a problem during gestation (an infection such as rubella for instance), infants can be born with congenital cataracts in isolated sections or layers of the lens (see Chapter 12). Until 8 weeks of gestation, the diameter of the lens is greater in the anterior-posterior direction than across the equator parallel to the iris. The lens is then almost spherical at birth with a reversal occurring during childhood so that in adulthood it is elliptically elongated parallel to the iris plane.
The optical characteristics of the lens, therefore, must mature significantly over the first months or years after birth (see the discussion of emmetropisation in Chapter 4).
The optical characteristics of the lens, therefore, must mature significantly over the first months or years after birth (see the discussion of emmetropisation in Chapter 4).
![]() Figure 1.5. Schematic of the lens vesicle, showing the future capsule and formation of the primary lens fibers. |
The optic cup is sitting behind the lens. One clinically important aspect of its development is its asymmetric invagination, which starts from the section that will form the inferior and nasal part of the eye. The most extreme invagination, including a part of the optic stalk, occurs there. The asymmetry results in the formation of a single, long embryonic fissure along the inferior nasal section of the cup and stalk (Fig. 1.6). The walls of the fissure should seal later in development, and a failure to do so results in a relatively rare condition seen in the clinic, coloboma.
The two walls of the cup are destined to become the retinal pigment epithelium and neural retina in the posterior part of the eye, and components of the iris and ciliary body in the anterior part of the eye. Another clinically important point that can be understood from the embryology of the posterior eye at this early point in gestation is that a retinal detachment, even in adults, occurs at the interface between the retinal pigment epithelium and the neural retina. The tissue separates at the point where the apical sides of the layers had folded to meet each other in development.
Formation of Support Tissues
The other tissues of the eye that support the function of the neural retina, lens, and cornea are typically derived from a third type of embryologic tissue, mesoderm (as compared with the neural and surface ectoderms). This tissue in the head and neck region is somewhat different from that in the rest of the body, because no mesodermal somites are found in the head region. This tissue in and around the eye consists of some conventional mesoderm, but is mainly derived from neural crest cells that have migrated to the eye and, by day 26, surrounded the optic vesicle between it and the surface ectoderm (Fig. 1.1B). This combination of tissues is denoted as ocular mesenchyme. The neural crest cells also form components of the teeth, middle ear bones, meninges, and endocrine glands, and so abnormalities in the development of these cells can result in congenital malformation of a number of these tissues (12).
The primary contribution of mesenchyme to the anterior eye consists of three waves of neural crest cell migration into the anterior chamber during the seventh week of gestation. Disruption of these waves is thought to lead to a number of clinical disorders of the anterior chamber (13). The first wave enters between the surface ectoderm and lens to form the initially multilayered corneal and trabecular endothelium. Another wave migrates from the periphery between the developing corneal epithelium and endothelium, to form the keratocytes that will become the corneal stroma, and the third wave forms the iris stroma.
The mesenchyme gains access to the posterior section of the eye through the embryonic fissure along the side of the optic cup and stalk (Fig. 1.6). This mesenchyme has the role of filling the optic cup with vitreous and providing this early posterior chamber with a blood supply. The future transparency of the cornea, aqueous, lens, and vitreous is critically dependent on the nature and spacing of their contents. The vasculature is able to supply these structures without disrupting their future transparency or permanently blocking the optical path to the retina. There are two principal stages to the formation of the blood supply. The first, the hyaloid system, is only present during gestation and typically degenerates before birth. It does, in fact, temporarily block the optical path to the retina and, therefore, would not be an efficient system to maintain after birth.
The hyaloid system is supplied by the terminal portion of the ophthalmic artery (a branch of the internal carotid), which passes through the embryonic fissure into the optic stalk. It runs along the lumen of the invaginated stalk and into the optic cup through the future optic nerve head. This artery is called the hyaloid artery. It grows forward through the vitreous, sending branches to meet the posterior surface of the lens. These branches then form a network of capillaries over the posterior surface of the lens, known as the posterior tunica vasculosa lentis. They also pass further forward to connect with the annular vessel forming around the rim of the cup. The annular vessel principally forms from vessels approaching along the cup’s outer surface (the ciliary arteries start to form around the outside of the optic cup during the seventh and eighth weeks). The annular vessel, in turn, sends loops forward to supply the anterior surface of the lens. These loops form the anterior tunica vasculosa lentis. This hyaloid system is fully formed by the end of the second month of gestation. The blood in this network reaches the venous system via vessels starting to form in the choroid (Figs. 1.3 and 1.4).
The development of the vitreous also consists of two sequential stages. The primary vitreous develops during the fifth week of gestation. It is derived from mesenchyme migrating in through the embryonic fissure and ectodermal fibers connecting the sensory retina to the posterior side of the lens. As with the hyaloid vasculature, the primary vitreous also degenerates to be replaced by a more mature secondary system. In the case of the vitreous, this secondary system starts to form almost as soon as the primary version appears—the end of the sixth week. The secondary vitreous forms at the sensory retina as a compact fibrillar network that does not blend with the primary vitreous at all, but gradually detaches it from the retina pushing it toward the hyaloid artery running from the optic disc to the lens.
The embryonic fissure starts to close and seal around the posterior chamber containing the new hyaloid vessels and vitreous in the sixth week. The closure starts about halfway along the side of the optic cup and progresses anteriorly and posteriorly from there. The last notch at each end closes during the seventh week. As mentioned, the fissure failing to close completely results in a coloboma (see Chapter 13).
The outer layer of the optic cup itself starts to differentiate into the retinal pigment epithelium (RPE), which will ultimately provide support to the photoreceptors and neural retina. By the fifth week, the RPE consists of two to three layers of cells that by the end of the week stop mitotic division and show all stages of pigment granule formation (in a similar pattern to that of the epidermal melanocytes) (Fig. 1.4A). The layer becomes the mature one cell thick during the sixth week, with apical projections toward the future photoreceptor outer segments (Fig. 1.4B). The basal lamina, meanwhile, starts to develop into Bruch’s membrane. The number and morphology of the RPE cells does not
change after approximately 8 weeks, although generation of melanosomes to form pigment continues until week 27 (14). The RPE, therefore, grows in area through expansion of the cells rather than by cell division in the later stages of gestation and postnatal period.
change after approximately 8 weeks, although generation of melanosomes to form pigment continues until week 27 (14). The RPE, therefore, grows in area through expansion of the cells rather than by cell division in the later stages of gestation and postnatal period.
The mesenchyme also starts to form structures around the outside of the optic cup during the second month. Outside the RPE, primitive vessels and stromal components of the choroid are visible in the mesenchyme during the fourth week of gestation. A vascular net of capillaries, derived from the dorsal and ventral ophthalmic arteries, becomes continuous over the whole cup in the fifth week. Formation of these vessels appears to depend on the development of pigment in the RPE. This net anastomoses with the annular vessel around the rim of the optic cup, and the hyaloid system within the cup. The connections with the vessels inside the cup are broken when the fissure seals, however. The blood passing through these capilliaries drains through a loose connection to two large plexuses, the supraorbital and the infraorbital venous plexuses. The first cells of the sclera are seen differentiating and condensing over the early choroid in the seventh week. They appear anterior to the equator of the cup first, then further forward toward the future limbus and more posteriorly, moving back toward the optic nerve.
The extraocular muscles that will move the eyes, to permit single binocular vision, first ap- pear as small, dense condensations of mesenchyme tissue. The muscles that will be innervated by the third cranial nerve appear at 26 days, the lateral rectus that will be innervated by the sixth cranial nerve appears at 27 days, and the superior oblique that will be innervated by the fourth cranial nerve first appears at 29 days. The third and sixth cranial nerves grow from the brain into these condensations at about 31 days, and the fourth cranial nerve follows at 33 days. The fibrous trochlea is seen at the end of the embryonic period of gestation (8).
The Fetal Period (9 Weeks to Birth)
The fetal period of development stretches from 9 weeks of gestation until birth at around 38 weeks. This period is characterized by the development of the function of ocular tissues and, therefore, disruptions during this period more often result in abnormalities of function rather than gross structure.
Cornea
At 8 weeks, the primitive epithelium has been formed from surface ectoderm, the endothelium from the first wave of mesenchyme, and the stroma from a later wave. The epithelium consists of outer squamous and basal columnar cells that sit on a basal lamina. Behind that, the stroma consists of 15 layers of cells with rapidly appearing collagen fibrils. Peripherally, it is blended with condensing mesenchyme that has spread back around the outside of the optic cup forming the developing sclera. By the end of the third month, the endothelium has formed into a single layer of cells, as seen in the adult, and it rests on a basal lamina that forms the first evidence of Descemet’s membrane. Hence, the only layer of the cornea missing at the beginning of the fourth month is Bowman’s membrane. This final layer, which appears during the fifth month, is acellular from this first stage. These corneal layers become more specialized over the next months.
Many nerve endings are distributed in the corneal epithelium by midterm. Also present are neurotubules and vesicles indicating stimulus transduction (15).
The final transparency of the cornea depends on the patterning and spacing of collagen fibers. These fibers are laid down over some weeks, parallel to the corneal surface, and both thicken and lengthen as the cornea grows. The early embryonic cornea is translucent, rather than transparent, and only becomes transparent at the time that the corneal water content is controlled and balanced in the fetal period (during this period, as in adulthood, the corneal stroma is hydrophilic and a metabolic pump is needed to remove water). Zonulae occludens only appear in the endothelium during the fourth month, but they tightly seal the endothelium just at the onset of aqueous humor formation. Ehler et al. (16) found the diameter of unfixed human corneas to grow from 2 mm at 12 weeks of gestation to 4.2 mm at 16 weeks, to 9.3 mm at 35 weeks of gestation (the diameter
then only increases by around 20% after birth). Collagen growth and replacement slow as the adult corneal diameter is reached.
then only increases by around 20% after birth). Collagen growth and replacement slow as the adult corneal diameter is reached.
Rapid growth of the cornea during the third month, relative to the rest of the eye, and the appearance of mesenchymal tissue in the limbal area both result in an increase in corneal curvature relative to that of the sclera (17). At birth, the optical power of the cornea is more than 50 D, significantly higher than that of the typical adult cornea (18,19,20). This has dramatic implications for the overall optical power of the neonatal eye (see the discussion of emmetropisation in Chapter 4).
Anterior Chamber
As development progresses into the fetal period, the space between the anterior section of the optic cup, the lens, and the cornea is filled with mesenchyme. The mesenchyme starts to form the structures of the future anterior chamber and angle. At this point, the vacuole forming the future chamber is much smaller than that found in adults and does not extend into the peripheral location of the future angle (Figs. 1.7 and 1.8). In fact, the structures that will drain the aqueous humor from the eye form in approximately the correct future location, but at this point are fully surrounded by mesenchyme. During the fourth month, the early trabecular meshwork consists of a triangular-shaped mass of undifferentiated mesenchymal cells with the anterior point forming between the corneal stroma and endothelium. The corneal endothelium, therefore, sits between the meshwork and the anterior chamber, unlike in the adult where the meshwork is open to drain aqueous from the anterior chamber (Fig. 1.7). Schlemm’s canal starts to form as a loose collection of pieces that then coalesce over the next 3 months. It is complete in at least some quadrants in the seventh month and completely formed in the ninth month (21,22). During the second half of gestation, the cells of the trabecular meshwork and Schlemm’s canal differentiate and form cavities to become more and more specialized for their role in draining and filtering the aqueous.
While the drainage system is maturing, the cells between the trabecular meshwork and ciliary body start to separate, allowing the anterior
chamber angle to extend more peripherally. The ciliary muscle and ciliary body are initially found adjacent to the trabecular meshwork, but as the angle opens they move posteriorly to expose the angle structures to the aqueous humor (Figs. 1.7 and 1.8). The trabecular meshwork is uncovered at around 7 months, but the ciliary body and iris continue to slide backward for approximately a year after birth (23).
chamber angle to extend more peripherally. The ciliary muscle and ciliary body are initially found adjacent to the trabecular meshwork, but as the angle opens they move posteriorly to expose the angle structures to the aqueous humor (Figs. 1.7 and 1.8). The trabecular meshwork is uncovered at around 7 months, but the ciliary body and iris continue to slide backward for approximately a year after birth (23).
Kupfer and Ross (24) and Pandolfi and Astedt (25) measured aqueous outflow in fetal eyes. They found it to be 0.09 μL/min/Hg before 7 months and 0.3 μL/min/Hg at 8 months.
Infants with significantly reduced aqueous drainage develop congenital glaucoma (see Chapter 13). A number of studies have examined tissue from these eyes and noted that the ciliary body and iris lay further forward than in a typical eye. These structures overlap the posterior part of the trabecular meshwork, resembling the developing angle of an infant at 7 or 8 months of gestation. Evidence is mixed of cases where the abnormality appears to result from premature cessation of development of the angle, a disruption of differentiation of the trabecular meshwork (26), or trabecular meshwork strands that are too thick and strong holding the angle shut (23). The trabecular meshwork and Schlemm’s canal are present in these eyes and surgery to open the angle is usually successful. Similar anterior chamber malformations can be seen in other, more extensive anomalies and syndromes (12). These conditions can result in secondary glaucoma for similar reasons. Currently, numerous studies are exploring the role of abnormalities in neural crest cell migration and terminal induction in these conditions. As mentioned, many of the affected children have abnormalities of other adjacent structures that are derived from the same cells (27,28).
Ciliary Body and Iris
The posterior wall of the anterior chamber is formed by the iris and ciliary body. The anterior section of the optic cup forms the bulk of these structures. The outer pigmented layer of the cup forms the ciliary epithelium, anterior iris epithelium, and the iris sphincter and dilator
muscles. The inner nonpigmented layer of the cup forms more ciliary epithelium and the posterior pigmented epithelium of the iris. By 12 weeks of gestation, the outer and inner layers of the optic cup are adhering to each other anteriorly, just behind the growing and advancing margin that will form the edge of the pupil and iris. This fused section of the optic cup starts to form 70 to 75 meridional ridges radiating out from the pupil. These are the precursors of the ciliary processes. The folding is thought to require the presence of the lens to induce differentiation. The next portion of the cup, on the peripheral side of the ciliary processes, forms a smooth section that will develop into the future pars plana.
muscles. The inner nonpigmented layer of the cup forms more ciliary epithelium and the posterior pigmented epithelium of the iris. By 12 weeks of gestation, the outer and inner layers of the optic cup are adhering to each other anteriorly, just behind the growing and advancing margin that will form the edge of the pupil and iris. This fused section of the optic cup starts to form 70 to 75 meridional ridges radiating out from the pupil. These are the precursors of the ciliary processes. The folding is thought to require the presence of the lens to induce differentiation. The next portion of the cup, on the peripheral side of the ciliary processes, forms a smooth section that will develop into the future pars plana.
Ozanics and Jakobiec (8
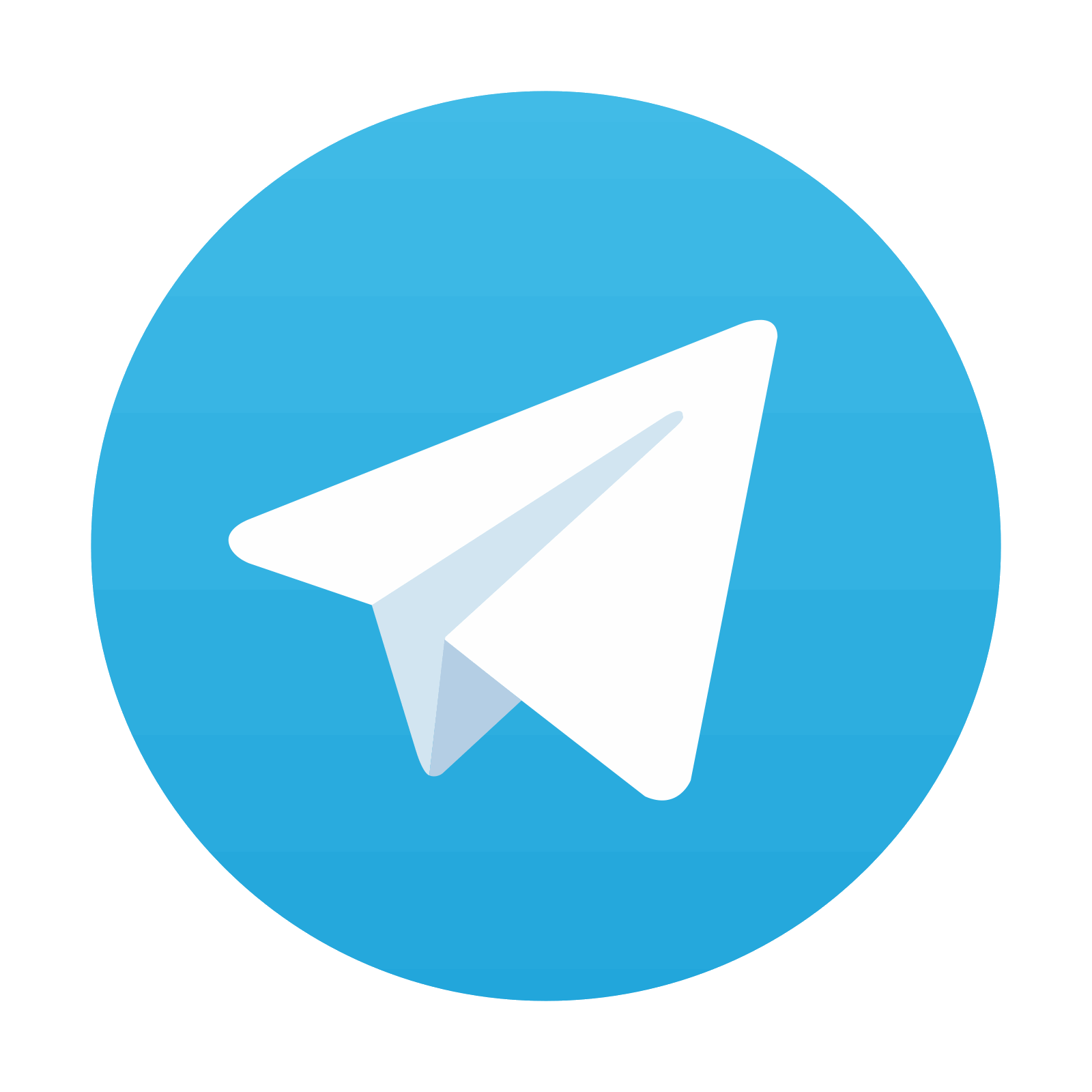
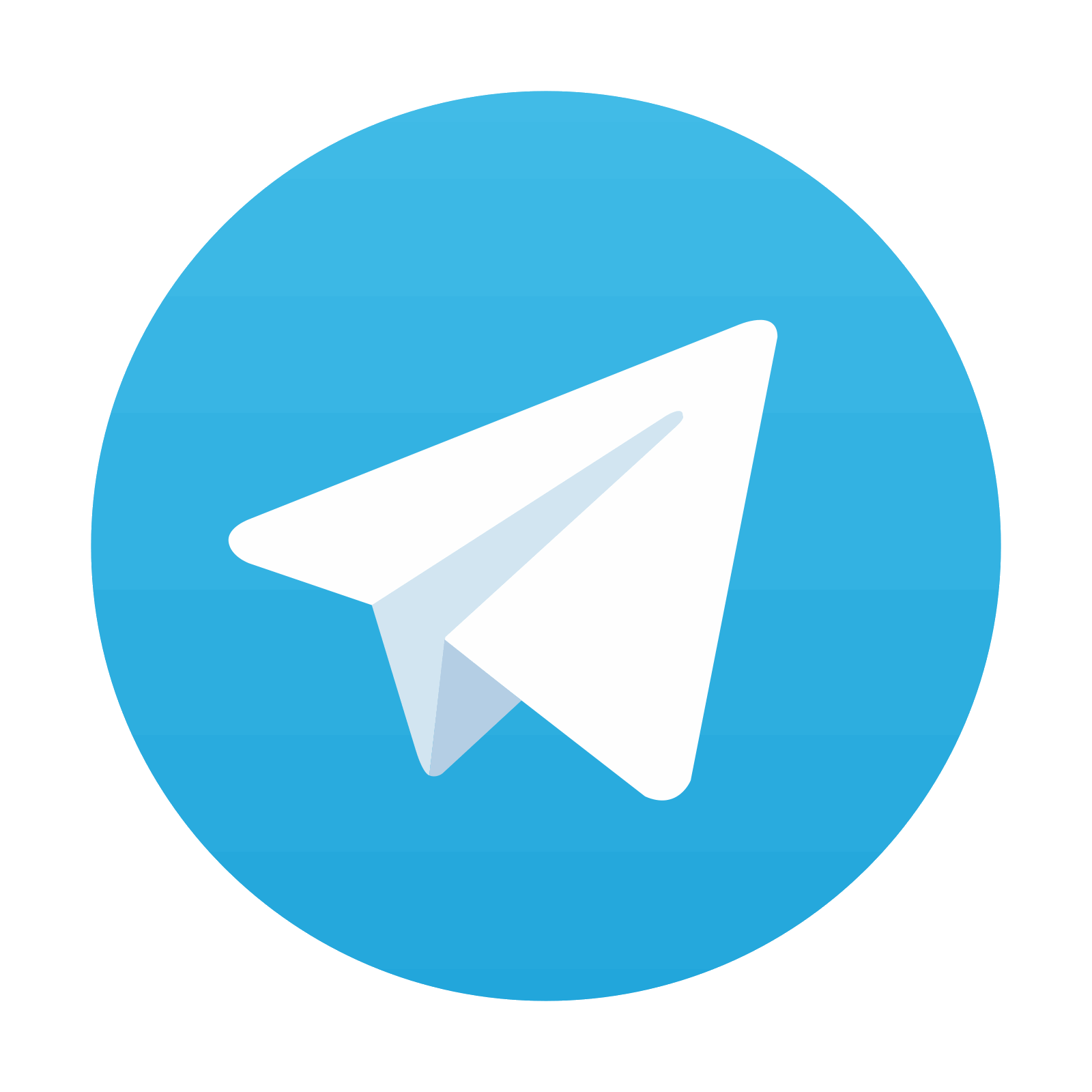
Stay updated, free articles. Join our Telegram channel
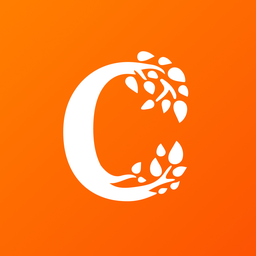
Full access? Get Clinical Tree
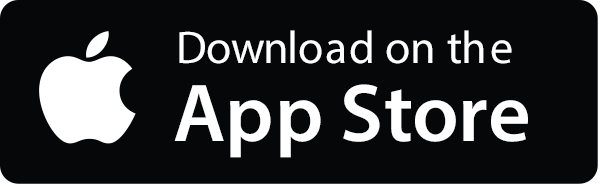
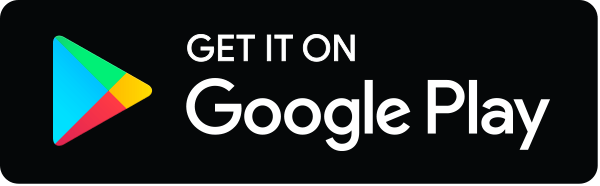
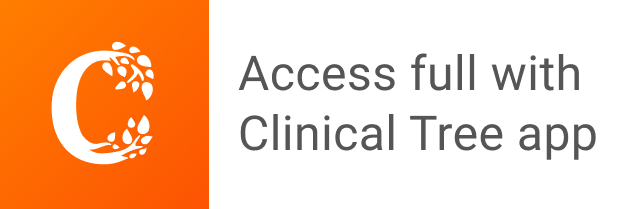