Corneal Physiology
Stephen D. Klyce
The cornea forms the transparent anterior portion of the ocular tunic, serving as the major refractive element in the eye while protecting its contents through unique physical and physiologic properties. Tissue transparency is rarely found in the animal kingdom outside of the eye, and the cornea and lens have served as models for understanding the structural and functional requisites for this property that conveys images from the environment to the photoreceptor layers in the retina. The cornea evolved as an avascular tissue, relying on the atmosphere for the bulk of its oxygen requirements and on the aqueous humor for most of its other nutritional needs. Being privileged through the constant moistening action of the tears spread by the blinking of the eyelids, the corneal surface normally maintains an optically smooth surface. Components of the tears derived from specialized cells in the conjunctiva and from the lacrimal gland further serve to protect the corneal surface from disruption and infection. The mechanical strength needed by the corneal stroma is provided by collagen fibers whose structure and organization is different from that of skin or the contiguous sclera. All connective tissues in the body rely on membrane active ion transport processes for the prevention of edema; the cornea, in particular, must rely on the metabolic activity of its outer stratified epithelium and inner monocellular endothelium for the maintenance of its state of hydration, for edema can lead to opacification and loss of vision.
The principal layers of the cornea are the epithelium, Bowman’s layer, stroma, Descemet’s membrane, and the endothelium (Fig. 2-1). This chapter concentrates on the fundamentals of corneal physiology to provide a foundation for understanding the normal condition as well as how corneal disease and trauma can upset corneal homeostatic regulatory processes, requiring medical and, sometimes, surgical intervention.
CORNEAL THICKNESS
One of the most useful and direct measures of corneal health is pachymetry; thin corneal areas can indicate keratoconus or other ectatic disease, whereas a thickened cornea usually relates to endothelial dysfunction. The normal adult human cornea obtains a nearly uniform thickness in its central 3 mm and thickens considerably in the periphery. Hence, in profile the posterior surface overall has a shorter radius of curvature (it is steeper in units of diopter) than the anterior surface. It is important to differentiate this natural, global feature of corneal posterior surface shape from posterior keratoconus, which usually confines itself to a localized area of decreased radius of curvature and consequent corneal thinning. The early measures of corneal thickness using manual optical pachometers reported an average central corneal thickness of 520 μm (1, 2, 3). However, the manual methods, although capable of good accuracy, remain cumbersome in routine clinical use, and automatic acoustic pachometers became the gold standard despite calibration uncertainties. Although the speed of sound is not well established in the living cornea, modern ultrasound pachometers generally yield average central corneal thicknesses of approximately 560 μm. Still, the most careful and recent optical studies place normal central corneal thickness at approximately 520 μm (4). Accurate measurement of corneal thickness has become relevant to clinicians because screening criteria for refractive surgeries have been established based on the lower limit of the range for normal thickness. With calibration factors among pachometers at variance and producing errors in accuracy of 40 μm or more, caution is suggested in the comparison of data obtained from different instruments.
THE CORNEAL STROMA
The bulk of the cornea is formed by the fibrous stroma, which in the human adult is approximately 470 μm thick centrally. Collagen fibers approximately 22 to 32 nm (5,6) in diameter appear to run uninterrupted from limbus to limbus in flat sheathes or lamellae. The lamellae are stacked at angles to one another and number in the hundreds in the human cornea. Unlike collagen fibers in most other
connective tissues, stromal collagen has a fairly uniform diameter, and in the bulk of the tissue does not branch at all. This is in contrast to collagen fibers in the sclera, which branch freely and whose diameters range from 25 to 480 nm. Corneal stromal fibers also exhibit a uniform center-to-center spacing of approximately 55 nm that is maintained by intramolecular forces in the surrounding extracellular matrix and by hydrational control mechanisms, which are discussed later.
connective tissues, stromal collagen has a fairly uniform diameter, and in the bulk of the tissue does not branch at all. This is in contrast to collagen fibers in the sclera, which branch freely and whose diameters range from 25 to 480 nm. Corneal stromal fibers also exhibit a uniform center-to-center spacing of approximately 55 nm that is maintained by intramolecular forces in the surrounding extracellular matrix and by hydrational control mechanisms, which are discussed later.
This organization of collagen fibers in the stroma is important for its optical characteristics, but the lack of significant interweaving and branching enhances the ability of the corneal stroma to swell. The extracellular matrix has a high content of hydrophilic glycosaminoglycans, notably chondroitin and keratin sulfates, which provide connective tissue with a great capacity to imbibe fluid when provided free access to it. Although the corneal stroma can swell significantly before light scatter from within it causes significant problems with vision, the mechanism of its transparency was a matter of conjecture for a considerable period of time.
The uniform distribution of collagen fibers in the stroma (Fig. 2-2) led Maurice to propose that transparency was achieved by a perfect crystalline lattice arrangement. He proposed that light scattered by individual fibers is cancelled by destructive interference with scattered light from neighboring fibers (7). However, Goldman and Benedek (8) and others (9,10) recognized that refractive elements in tissues whose dimensions are small (<200 nm) compared with the wavelength of light (˜500 nm) should not scatter as much light as might be predicted by the stringent requirements of the crystalline lattice theory. Consequently, the normal stroma scatters light minimally because its collagen fibers are uniformly small in diameter and closely spaced. Furthermore, even the quasiregular arrangement of stromal collagen fibers appears to be an unnecessary requirement for transparency. In the human, Bowman’s layer is 8 to 12 μm thick and its constituent collagen fibers are arranged irregularly; nevertheless, it is relatively transparent. According to the Goldman-Benedek criterion, connective tissue should remain relatively transparent as long as the spatial dimensions of variations in refractive index remain less than 200 nm. As the stroma swells, fluid entry expands the ground substance, which increases the spacing between collagen fibers. As the spacing increases, the orderliness of the fibers decreases, and light scatter ensues when the fluctuations in refractive index exceed the Goldman-Benedek criterion. This criterion also applies to the origin of light scattering in the human corneal epithelium, where intracellular fluid collection causes large spatial fluctuations in refractive index (11). Although the physical basis for transparency of the stroma and epithelium is identical in this respect, stromal edema and epithelial
edema are considered separately later because the pathophysiologic processes and clinical effects are somewhat different.
edema are considered separately later because the pathophysiologic processes and clinical effects are somewhat different.
![]() FIGURE 2-3. The keratocyte nuclei in Bowman’s layer are highly refractile on confocal microscopy in this normal human cornea. |
The Cells of the Stroma
The cellular density in the stroma is low in keeping with the optical characteristics of this tissue. Each stromal cell scatters light, as slit-lamp or confocal microscopic examination reveals (Figs. 2-3 and 2-4), but the total light scattered by the stroma at normal hydration is small, permitting most light to be transmitted. The numerous keratocytes occupy 3% to 5% of the volume of the stroma and are distributed throughout the stroma, with their flattened cell bodies lying primarily between lamellae. Keratocytes extend processes outward from their cell bodies between the collagen lamellae, and the tips of these processes sometimes touch those of a neighboring keratocyte and form a tight junction. The tight junctions are a form of cell-to-cell communication and are thought to be important in the regulation of keratocyte function (12). Nerve axons and their associated Schwann cells are found in the anterior and middle thirds of the stroma. Each of these very different cell types plays important roles in maintaining the health and function of the cornea.
Role of the Keratocytes
Keratocytes maintain the collagen scaffold and extracellular matrix of the stroma. Collagen constitutes approximately 71% of the dry weight of the cornea (13,14), and in the quiescent cornea, both procollagen and collagen are secreted at a low basal rate. However, keratocytes can become activated by surgery or trauma, initiating a wound-healing response that invokes greatly increased collagen secretion (15).
Keratocytes undergo extensive cellular alteration in response to corneal injury, particularly in regions proximal to damaged epithelium. In response to an epithelial scrape, keratocytes are depleted rapidly from the subjacent corneal stroma (16). The mechanism of this response has been studied extensively (17,18). Injury to the corneal epithelium causes the release of cytokines to the stroma, which in turn stimulates programmed cell death (apoptosis) of the underlying anterior stromal keratocytes. It is hypothesized that proteolytic enzymes are also released during apoptosis, causing a local breakdown of stromal macromolecules that increases the stromal colloid osmotic pressure and leads to localized edema (19,20). It is thought that the localized edema that characterizes the acute phase of the injury response in all tissues provides an avenue of greater mobility for activated keratocytes and, in some cases, inflammatory cells in their migration to the wound site. When the stroma is repopulated with activated keratocytes, these cells can produce collagen and other components associated with stromal remodeling. In addition, activated keratocytes can secrete hepatocyte growth factor and keratinocyte growth factor, which can stimulate proliferation and inhibit differentiation of epithelial cells (21). When this occurs, epithelial
hyperplasia can result, with alteration of the corneal surface curvature and an associated change in refractive error that can cause a variable outcome after refractive surgical procedures.
hyperplasia can result, with alteration of the corneal surface curvature and an associated change in refractive error that can cause a variable outcome after refractive surgical procedures.
Stromal Transport Properties
The corneal stroma is primarily an extracellular compartment, with keratocytes and nerves accounting for only 5% and 0.01% of its volume, respectively. Although the concentrations of K+ and Na+ are 35 mEq/L higher in the stroma than in the aqueous humor (22), their effective concentrations (activities) are reduced by physicochemical factors such as ion binding by anionic sites on stromal macromolecules. This makes the chemical activities of the major diffusible micro-ions (K+, Na+, and Cl−) similar in the stroma and the aqueous humor. This feature is central to the concept of osmotic control of corneal stromal hydration, discussed later.
The negative charges of glycosaminoglycans (10 to 50 mEq/L) are primarily responsible for the abundance of Na+ and K+ in the stroma (23, 24, 25). When the stroma swells, the diameter of the collagen fibrils remains essentially constant; swelling takes place in the ground substance, which is rich in glycosaminoglycans, and leads to an increased spatial separation of the collagen fibrils (26). Several factors influence the swelling force generated by the ground substance: long-range electrostatic repulsive forces between negative charges on the glycosaminoglycans, excess cations required to preserve ionic charge neutrality (Donnan effect), mechanical limitations in the corneal structure that prevent swelling beyond a certain limit (27), and chemical effects such as changes in pH and total ionic strength. For corneal stroma, the major forces normally involved in stromal swelling appear to be long-range electrostatic repulsion and the Donnan effect (14).
In addition to imparting the underlying force for stromal swelling, the glycosaminoglycan component of the stromal ground substance has other effects on the nature of stromal swelling. Glycosaminoglycans form polymeric macromolecules, which in turn associate with protein cores to form megamolecular complexes in the ground substance. These complexes increase the viscosity of the fluid in the ground substance in a stromal hydration-dependent fashion. Fatt and Goldstick (28) pointed out an important feature of fluid flow in tissues such as stroma: The ability of fluid to flow (to even out hydration gradients) is greatest in tissue that has near-normal hydration and less for swollen or dehydrated tissue, which helps preserve homeostasis in the internal environment. For example, as the stroma is dehydrated below normal conditions, the driving force for fluid flow (swelling pressure gradient) increases exponentially, whereas the stromal hydraulic fluid conductivity decreases as a power function of hydration. Because of these properties, normal corneal thickness is responsive to subtle changes in the environment, as demonstrated by the 5% thinning during waking hours, yet local dry spots or dellen can persist in the anterior stroma while the surrounding tissue is normally hydrated.
The stroma is normally maintained in a relatively dehydrated state compared with its ability to swell. The stroma consists of 78% water, which is equivalent to a ratio of 3.45 parts water (by weight) to 1 part solid material. Thus, the hydration is 3.45. The ability of the stroma to swell decreases as its hydration increases. This relationship has been carefully measured for corneal stroma by placing disks of stroma between layers of rigid, porous glass in a saline solution. The force necessary to prevent stromal swelling at various hydrations has been termed the swelling pressure (SP); these measurements show a near-exponential drop with increasing stromal hydration (Fig. 2-5). At normal hydration (3.45), the stromal SP is approximately 80 g/cm2 or 55 mm Hg (29,30). This is the amount of force that corneal membranes must create to counterbalance stromal swelling in the living cornea, and is equivalent to a stromal osmotic deficit of 2 to 3 milliosmoles (mOsm).
It has been proposed that the SP of stroma as measured in vitro is not an adequate measure of the situation in the living cornea. It was suggested that measurements of SP
determined with excised stroma could be misleading because the conditions normally present in the living eye have been altered. However, Hedbys and coworkers (31) implanted fine saline-filled cannulas into the central stroma of living rabbits and showed that a negative pressure in the cannula was necessary to prevent the stroma from drawing fluid continuously from the tube. The equilibrium suction that prevented this fluid loss was termed the stromal imbibition pressure (IP) and was found to be related to the stromal SP by the simple relation:
determined with excised stroma could be misleading because the conditions normally present in the living eye have been altered. However, Hedbys and coworkers (31) implanted fine saline-filled cannulas into the central stroma of living rabbits and showed that a negative pressure in the cannula was necessary to prevent the stroma from drawing fluid continuously from the tube. The equilibrium suction that prevented this fluid loss was termed the stromal imbibition pressure (IP) and was found to be related to the stromal SP by the simple relation:

In the absence of intraocular pressure (IOP) effects, the IP measured in vivo was identical to the SP measured in vitro. Because the tension of the IOP is taken up principally by that anterior corneal lamellae, this hydrostatic pressure diminishes the measured IP.
The pressure of swelling in the normal cornea in vivo was confirmed by a second approach by Klyce and colleagues (32), who inserted thin, high-water-content hydrogel disks into intralamellar stromal pockets and found that, after the eyes stabilized, the disks were thinner by the amount previously predicted on the basis of stromal SP. The in vivo cannulation and gel implant experiments confirm the notion that the tendency of the stroma to swell in vivo is the same as was indicated by earlier in vitro experiments.
Ytteborg and Dohlman (33) explored the significance of equation [1], and showed that when the IOP exceeds the SP, the IP turns positive and subepithelial edema ensues. This dynamic relationship has clinical implications. Stromal SP is normally 50 to 60 mm Hg; the IOP in glaucoma rarely exceeds this value. However, as noted previously, stromal SP drops off exponentially with stromal edema, so that mild stromal edema combined with mild IOP elevation can lead to positive stromal IP, which, because of the barrier properties of the epithelium, can lead to the collection of intraepithelial fluid. Figure 2-6 relates the appearance of epithelial edema to IOP and corneal thickness.
The stromal experiments led to another important topic in stromal physiology, which deals with how IOP distributes itself across corneal thickness in terms of stress factors on the structural tunic. This has an important bearing on current problems in refractive surgery (34). IOP normally commutes through the stroma and is dissipated by stress on the anteriormost stromal lamellae. Several implications of this concept are discussed later. It is, however, important to emphasize that when anterior stromal lamellae are cut, they can no longer bear the stress of IOP, which is then transferred to the deeper, intact lamellae (34).
There is a linear relationship between central corneal thickness (q), expressed in millimeters, and stromal hydration (H), expressed as the ratio of the weight of water in the tissue to the dry tissue weight:

It has been shown experimentally that the hydration of the anterior stroma is less than that of the posterior stroma (35). This is usually taken to mean that there are differences in the types or concentrations of glycosaminoglycans at these different locations. However, such a gradient can be predicted by computer simulation on the basis of dissimilarities
in the permeability properties between the epithelium and endothelium alone, even in the absence of tear film evaporation (36).
in the permeability properties between the epithelium and endothelium alone, even in the absence of tear film evaporation (36).
The stroma requires continuous maintenance by its keratocytes to sustain its macromolecular composition and consequent organization. Normally, keratocytes, while having all the anatomic features consistent with synthetic activity, appear to be quiescent in terms of mobility. In fact, however, keratocytes are quite responsive to changes in their environment. They can mobilize to repopulate stromal areas devoid of keratocytes, and may rapidly undergo apoptosis below areas where trauma to the epithelium has occurred, as noted previously.
THE CORNEAL LIMITING CELL LAYERS
The corneal stroma is bounded by two cell layers with distinctly different structural and functional characteristics. On the outer surface a stratified layer of epithelial cells isolates the cornea from tearborne pathogens, providing a high-resistance barrier. The epithelial cells also provide marked regenerative properties to heal surface injuries rapidly through mitotic activity and migration. By contrast, the inner surface of the stroma is lined with a monocellular layer of fragile, highly permeable cells that show extremely limited regenerative capability after birth. But the inner cell layer, the corneal endothelium, is critical for the maintenance of normal corneal thickness through its membrane active ion transport processes that provide a homoeostatic mechanism to prevent stromal edema.
CORNEAL EPITHELIUM
The epithelium of the cornea consists of four to six layers of cells and represents 10% or approximately 55 μm of the corneal thickness (37). The three layers (squamous cell, wing cell, and basal cell) are coupled tightly by junctional complexes forming a functional syncytium through which electrochemical cross-talk can occur. The basal cells are the only epithelial cells that undergo mitosis. The daughter cells move anteriorly to the surface, maintaining communication with neighboring cells until desquamating into the tear film.
Epithelial Cell Turnover
The epithelium turns over approximately every 7 days (38). Limbal stem cells also provide a constantly renewing source for the corneal epithelium as they continually divide and migrate centripetally toward the corneal center in a vortex pattern. They move inward at a rate of 20 to 30 μm/day, reaching the center in approximately 7 weeks in the mouse (39). The stem cells that arrive at the center of the cornea are the progeny of those cells arising at the limbus. This constant renewal process is apparently necessary for homeostasis of the epithelium because limbal stem cell deficiency can cause reepithelialization by the neighboring conjunctival epithelium, vascularization, and recurring epithelial defects. Whether these limbal cells are true stem cells or “committed progenitors” is being debated (40); nevertheless, their contribution to the epithelium is essential.
Superficial Epithelial Cells
An important property of the superficial cell layer is the junctional complex formed with laterally adjacent cells. This complex consists of tight junctions that completely encircle the cell (41). The joining of adjacent superficial cells at their lateral borders in this manner prevents the movement of substances from the tear film into the intercellular spaces of the epithelium.
The apical tight junctional complex in combination with the very low permeability of the outermost membrane of the superficial squamous cell is the anatomic representation of the barrier property of the corneal epithelium, as demonstrated in electrophysiologic studies (42). Sensory nerve terminals in the epithelium help preserve the integrity of this important surface barrier structure by eliciting a severe pain response to injury. A clinical test to determine if this barrier is intact uses dyes, such as fluorescein, which are instilled into the conjunctival sac. The normal epithelial surface stains little or not at all. Rubbing the corneal surface under the closed eyelid damages the fragile surface cells, resulting in punctate staining (43). Diabetic patients have increased surface staining, which suggests that the barrier property of the epithelium is impaired (44). Several factors, such as rapid or abnormal epithelial cell loss or the inability to form tight junctions, could lead to surface staining in diabetes.
Wing Cells
The wing cell layer is formed by the transition and anterior migration from the basal cell layer. The wing cells interdigitate extensively and are interconnected by desmosomal junctions and gap junctions that serve as electrochemical conduits. This conductance extends to the squamous cells and to a lesser degree to the basal cells, and facilitates cell-to-cell communication. In this way, a pathway is provided for micro-ion transport in the commission of cell volume regulation.
Basal Cells
The cytoplasm of the basal cell has large numbers of glycogen granules, which represent a source of stored metabolic energy to be used in times of epithelial stress, such as hypoxia
and wound healing. Actin filaments are seen along the cytoplasmic side of the membrane, and may be important for cell migration in the healing of abrasions, during which these cells may become motile (45).
and wound healing. Actin filaments are seen along the cytoplasmic side of the membrane, and may be important for cell migration in the healing of abrasions, during which these cells may become motile (45).
CORNEAL TRANSPORT PROPERTIES
At the heart of corneal homeostasis are the membrane transport and permeability properties of the epithelium and endothelium as well as the fluid flow characteristics of the corneal stroma. The individual properties of these three layers are presented, followed by a discussion of a holistic model for the control of corneal hydration and transparency.
Epithelial Transport Properties
The primary function of the corneal epithelium with respect to corneal homeostasis is that of a barrier restricting both the entry of fluid as well as pathogens. Nevertheless, the corneal epithelium possesses active ion transport systems under neural control that also play a role in maintaining corneal function.
Active Na+ Transport in the Epithelium
Corneal ion transport systems have been intensely studied since it was shown that cooling the tissue, which slows down metabolism, produces edema. A tears-to-stroma transport of Na+ has been demonstrated in the rabbit and frog (46, 47, 48, 49, 50, 51, 52). This absorptive transport of Na+ presents somewhat of an anomaly inasmuch as an inward solute transport to the stroma would increase its osmotic pressure and presumably favor stromal edema. It has been proposed (53,54) that an increase in the stromal content of Na+ might neutralize the anionic groups of the glycosaminoglycans and reduce or prevent their ability to swell. However, in both rabbits (51) and humans (55), when factors such as corneal resting potential and other ion transport systems are taken into account, the net flow of Na+ (and Cl−) across the epithelium is from stroma to tears (56).
Nevertheless, the active Na+ transport system in the epithelium is powered by the specific energy-supplying enzyme for Na+ transport, Na+/K+-adenosine triphosphatase (ATPase), which has been demonstrated histochemically (57). The activity of this enzyme can be enhanced severalfold by reacting the superficial epithelial cell membrane with amphotericin B or Ag+ (58,59). Normally, the superficial epithelial cell membrane restricts the entry of Na+. However, both amphotericin B and Ag+ create cation channels in this membrane, which greatly increases intracellular Na+ concentration and the rate of absorptive Na+ transport. In this situation, the stroma swells in accord with the added osmotic load (59). The epithelial Na+ transport system is inhibited by ouabain, a specific inhibitor of Na+/K+-ATPase.
The transepithelial transport of Na+ is most likely secondary to the K+/Na+ exchange activity of the deeper epithelial membrane, which maintains the high K+ and low Na+ characteristic of the cells. The Na+/K+-ATPase activity of the epithelial surface is less than that of the basolateral membranes, which accounts for the directionality of transepithelial Na+ transport. The small amount of Na+ that leaks into the cells across the apical membrane is more efficiently removed by the basolateral membrane K+/Na+ exchange mechanism.
Active Cl− Transport in the Epithelium
The corneal epithelium also transports Cl− in a secretory direction, that is, from stroma to tears (49,60,61). Corneal epithelial transport of Cl− is regulated by the β-adrenergic receptor/adenylate cyclase complex. Stimulation of Cl− transport is accompanied by an elevation of intracellular cyclic adenosine monophosphate (cAMP) and can be accomplished by the addition of membrane-permeable forms of cAMP (49). Catecholamines, such as epinephrine, stimulate Cl− secretion, unless blocked by β-adrenoreceptor antagonists, such as propranolol or timolol. This stimulation is enhanced by theophylline, which is a compound that inhibits phosphodiesterase and slows the breakdown of cAMP.
Net Na+ absorptive transport and net Cl− secretory transport can occur simultaneously only under special experimental conditions, such as are found with the short-circuit current technique. In the living eye, the epithelium generates an electrical potential of approximately 30 mV, tear side negative, and in this situation the net movement of NaCl across the epithelium appears to be in the stroma-to-tears direction (51).
Microelectrodes have been used to analyze the site of action of epinephrine in the stimulation of Cl− transport. In the transport of ions across epithelial cells, there is an energy-consuming translocation of the transported ion at one epithelial surface and a passive diffusional translocation of the same ion at the opposite surface. In the rabbit cornea, the active step in Cl− transport appears to be Cl− uptake by the epithelial cells from the stroma in conjunction with a passive diffusional barrier at the corneal surface, which limits the exit of Cl− from the superficial epithelial cells to the tear film. The action of epinephrine in the regulation of the overall net secretion of Cl− appears to be the formation of functional Cl− conductance channels in the apical membrane of the superficial epithelial cells (62).
The presence of active Cl− secretory transport in the epithelium raises the possibility that the epithelium might participate in the regulation of stromal hydration in addition to its role as a diffusion barrier. In the frog, Cl− secretion by the epithelium produces significant stromal dehydration
(63,64). Epithelial fluid secretion has also been identified in the rabbit (65), where stromal thinning at a rate of 1.3 μm/hour is observed. Epithelial fluid transport in the primate has not been reported, although, as mentioned previously, Cl− secretion has been demonstrated.
(63,64). Epithelial fluid secretion has also been identified in the rabbit (65), where stromal thinning at a rate of 1.3 μm/hour is observed. Epithelial fluid transport in the primate has not been reported, although, as mentioned previously, Cl− secretion has been demonstrated.
The presence of catecholamine-activated β-adrenergic receptors as a control mechanism for epithelial function has been confirmed in a number of studies. The population of these receptors is dynamic; their density decreases after topical administration of epinephrine (66) and increases after superior cervical ganglionectomy (67), a manipulation that would be expected to deplete the normal supply of corneal catecholamines. Other sources of catecholamines, such as the tears and the aqueous humor, that might activate corneal β-adrenergic receptors have been considered, but concentrations of epinephrine in these compartments are minute, owing in part to the cellular activity of monoamine oxidase, the principal enzyme responsible for the catabolism of catecholamines. In addition, anatomic evidence presented earlier underscores the role of sympathetic fibers as the likely physiologic source of catecholamines in the cornea.
Other epithelial regulatory receptors have been identified, again using Cl− transport as a measure of functional response. Both serotonin (68) and dopamine (69) stimulate Cl− secretion in the rabbit cornea through mechanisms inhibited by specific receptor antagonists. Serotonin increases cAMP in the epithelium (70) and evokes membrane conductance changes similar to those of epinephrine, including increased Cl− conductance in the outer membrane of the superficial cells (71). The specificity of the serotonin and dopamine responses became suspect when it was found that timolol blocks their activity, especially because timolol has been found to cross-react with other receptor types, although it is nonselective, and blocks both β1– and β2-adrenoreceptors. In addition, it was shown that the corneas of animals that had undergone superior cervical ganglionectomy (67) and pure subcultures of corneal epithelium (72) lack responsiveness to serotonin. On this basis, it was suggested that the serotonin receptors and, potentially, the dopamine receptors are located on the sympathetic fibers themselves. In this scheme, activation of serotonin or dopamine receptors located on the sympathetic fibers causes the release of catecholamines (presumably norepinephrine), which, in turn, activates epithelial β-adrenoreceptors. Such a mechanism explains the apparent anomalous timolol antagonism to the serotonin response, as well as the disappearance of serotonin receptors in epithelial subculture. It does not alter the earlier notion that the β-adrenoreceptors are located on the epithelial membranes themselves, in that the responses to catecholamines in cultured cells remain intact (72). A summary of membrane ion transport in the corneal epithelium and its putative neural control is presented in Fig. 2-7.
This section has emphasized the neuropharmacology of specific corneal membrane receptors to detail a model for a sequence of events linked to epithelial Cl− transport, which, on its own merit, provides some understanding of epithelial function. However, activation of the β-adrenoreceptor increases cell levels of cAMP, which is a rather common second messenger in terms of cell signaling, and the assay of one specific function (Cl− transport) may reveal only one aspect of receptor activation in this tissue. Other functional modalities are likely to be controlled by this receptor, such as cell proliferation (72) during epithelial repair, corneal immunologic response, and recurrence of herpes simplex viral disease (73,74).
Barrier Function
One of the most useful clinical tools in the assessment of the barrier properties of the corneal epithelium is the topical application of fluorescein. Normally, the corneal epithelium is impermeable to this anionic molecule. As a result, normal areas stain little or not at all, whereas areas with epithelial defects stain intensely. The location of this barrier and the membrane events associated with the corneal epithelial transport systems have been examined by means of intracellular measurements of electrical potential and ion activities with microelectrodes.
Intracellular microelectrode recordings in the corneal epithelium have been reported in frogs, rabbits, and cats (37,42,59,62,75, 76, 77, 78, 79, 80, 81). Such work has led to several general conclusions, which are summarized in Fig. 2-8. As with other cells, corneal epithelial cells have negative intracellular potentials, which are an expression of the fact that most cells maintain a high ratio of K+ to Na+ by means of the ubiquitous K+/Na+ exchange pump, which assists in the regulation of individual cell volume. The negative intracellular potential is also related to the relatively high K+ permeability of most cell membranes. In such a situation, K+, which is favored to diffuse out of the cell, creates a Nernst diffusion potential across the cell membrane oriented so that the cell interior remains electrically negative.
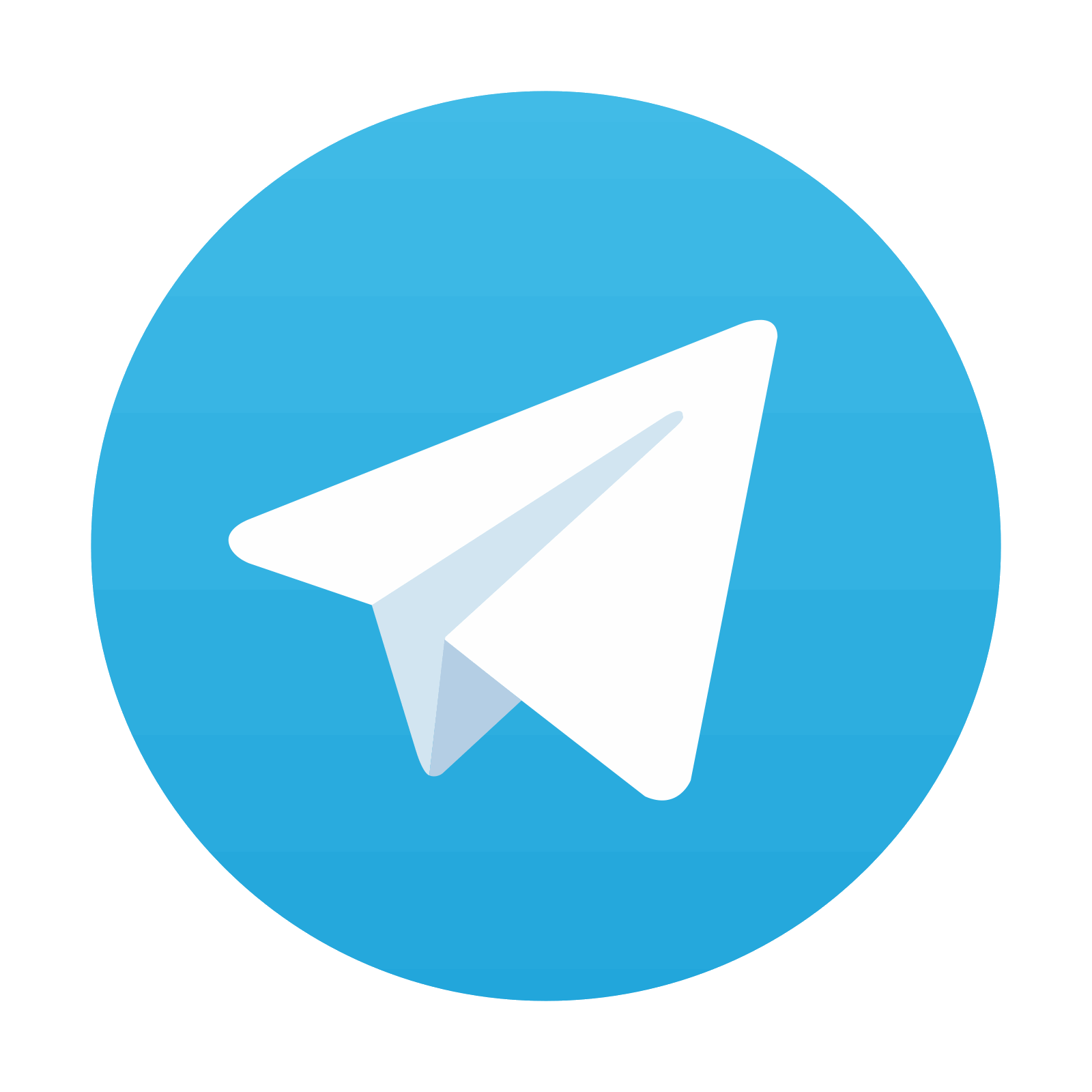
Stay updated, free articles. Join our Telegram channel
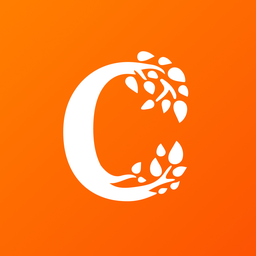
Full access? Get Clinical Tree
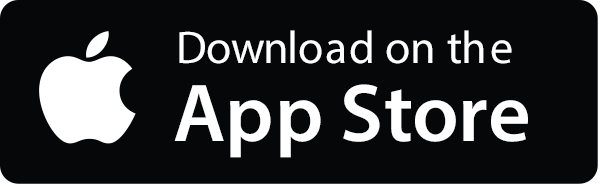
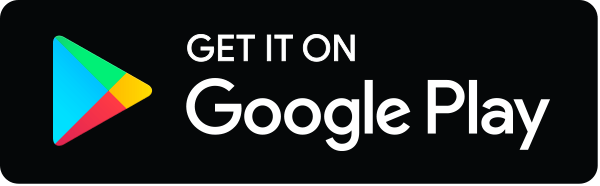
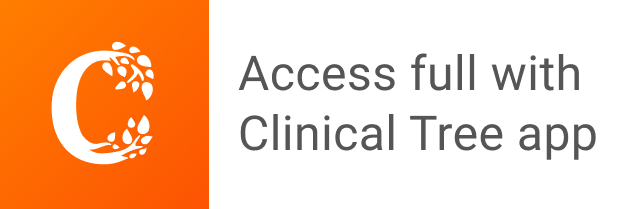