Overview
The corneal stroma accounts for 90% of the corneal thickness. The corneal stroma is predominantly composed of water (78% water or 3.5 g H 2 O/g dry weight). Its dry weight is organized into a structural network of insoluble and soluble cellular and extracellular proteins: collagen (68%), keratocyte constituents (10%), proteoglycans (9%), and salts, glycoproteins, or other substances. It is optically clear or transparent due to its lattice-like arrangement of small-diameter collagen fibrils and the near invisibility of its cells.
Although other cell types do exist in the cornea (e.g., Langerhans and dendritic bone marrow-derived immune cells, trigeminal nerve dendrites, Schwann cells, and histiocytes), the human cornea is primarily composed of three cell types: epithelial cells, stromal keratocytes, and endothelial cells. They can all replicate through mitosis, but they vary significantly in their in vivo self-mitotic capacity (proliferative capacity), with epithelial cells being the most renewable, stromal keratocytes in the middle, and endothelial cells the least renewable. The limited proliferative capacity of human corneal endothelial cells is apparently only an in vivo phenomenon as endothelial cells can proliferate quite well in ex vivo cell culture conditions. The in vivo mitotic quiescence of human corneal endothelium has been found to be predominantly due to cell contact inhibition, in part through the activity of p27. This results in corneal endothelial cells being arrested in the G 1 -phase of the cell cycle. High aqueous humor concentrations of transforming growth factor (TGF)-ß 2 , age-related cellular senescence, and lack of an injury-inducible cytokine-stimulating pathway are also secondary failsafe mechanisms that keep in vivo endothelial cell proliferation in check, if cell-to-cell contact is compromised. These facts about corneal cellular proliferative capacity can be seen clinically since epithelial cells can completely regenerate after injury (e.g., corneal abrasions) or can develop into cancer (e.g., squamous cell cancers that originate from limbal progenitor cells, or stem cells, at the palisades of Vogt). On the other hand, age-related (e.g., Fuchs’ dystrophy) or injury-related (e.g., pseudophakic bullous keratopathy) disease most commonly affects the corneal endothelium since it has little in vivo proliferative capacity.
The purpose of this chapter is to describe the pathophysiology of corneal edema.
Clinical background
Corneal edema is a term often used loosely and sometimes nonspecifically by clinicians, but literally refers to a cornea that is more hydrated than the normal 78% water content ( Box 9.1 ). With minor (<5%) hydration changes, the corneal thickness changes with minimal effect on the retractive, transparency, and biomechanical functions of the cornea. Only when the cornea become hydrated >5% above its physiologic level of 78% does it begin to scatter significant amounts of light and gradually loses transparency. Some loss of retractive function may also occur, particularly if the epithelial surface becomes irregular. The topic of corneal edema is important for clinicians to understand because it affects the architecture and function of the entire cornea. Epithelial edema clinically causes a hazy microcystic appearance to occur in the epithelium in mild-to-moderate cases of corneal edema ( Figure 9.1A ), significantly decreasing vision, and increasing glare. It can also cause the development of large painful, subepithelial bullae in severe cases of corneal edema ( Figure 9.1B ). Stromal edema clinically appears as a painless, cloudy, thickening of the corneal stroma ( Figure 9.1B ), resulting in a mild-to-moderate reduction in visual acuity and an increase in glare. At the same time, Descemet’s membrane folds commonly appear on the posterior surface of the cornea, particularly in severe cases of corneal edema ( Figure 9.1B ).
- •
Hydration > 78%
- •
Epithelial edema
- ○
Microcystic appearance (reduces vision and increases glare)
- ○
Bullae (sometimes very painful and causes epithelial erosions)
- ○
- •
Stromal edema
- ○
Cloudy thickening (reduces vision and increases glare)
- ○
Descemet’s membrane folds (reduces vision)
- ○

The exact incidence of corneal edema is unknown and is difficult to quantify since it is due to many causes and can fluctuate during the day or be transient or permanent in nature.
Clinically, the diagnostic workup includes slit-lamp examination and, commonly, pachymetry and/or specular or confocal microscopy to confirm whether corneal edema is present and to measure to what degree ( Box 9.2 ).
The differential diagnosis of corneal edema includes corneal scarring, corneal inflammation, corneal infection, and corneal dystrophies.
The treatment options include limited temporary medical options, such as topical salt drops (e.g., Muro) to remove the excess water from the cornea via osmosis, hair drier blowing on the cornea to induce increased evaporation, or topical steroids to increase endothelial cell tight junctions temporarily, and surgery (Descemet’s membrane-stripping endothelial keratoplasty, penetrating keratoplasty) to replace the damaged or deficient number of remaining endothelial cells ( Box 9.3 ). The main complications of surgery are graft rejection (endothelial cell allograft rejection), graft failure (transplanted endothelial cells are damaged by surgery or decrease to below optimal cell densities, usually because of chronic ongoing cell loss that is higher than normal unoperated eyes), decreased vision (irregular astigmatism, high astigmatism, or interface irregularity), infection (suture infections, ocular surface disease), and wound-healing issues (graft dehiscence, graft neovascularization, graft haze, neurotrophic epitheliopathy).
Treatment
- •
Medical adjuncts
- ○
Increase evaporation (hair drier)
- ○
Remove excess water with hyperosmotic agents (Muro eye drops)
- ○
Enhance tight junctions (topical steroids)
- ○
- •
Surgery
- ○
Full-thickness replacement (penetrating keratoplasty)
- ○
Component replacement (Descemet’s membrane-stripping endothelial keratoplasty)
- ○
Pathology
Epithelial edema is seen histopathologically as hydropic basal epithelial cell degenerative changes and the development of extra-epithelial cellular fluid-filled spaces (e.g., cysts and bullae: Figure 9.2A ). Interestingly, if bullae are chronically present, a fibrocollagenous degenerative pannus oftentimes will grow into the subepithelial space, decreasing vision further, while reducing the pain. Histopathologically, the signs of stromal edema are seen on the light microscope as thickening of the corneal stroma in the posterior cross-sectional direction with loss of artifact stromal clefting ( Figure 9.2A ) and Descemet’s membrane folds ( Figure 9.2B ). Ultrastructural and biochemical studies have further shown that stromal edema causes hydropic degenerative changes or cell lysis to occur in the resident keratocyte population ( Figure 9.2B and C ), an increase in the distance and disruption of spatial order between collagen fibrils ( Figure 9.2C inset), a decrease in the refractive index of the extracellular matrix, and a loss of proteoglycans. The hydropic degenerative changes of the keratocyte and possibly the intrafibrillar lakes of fluid are the main correlates for the stromal cloudiness resulting from corneal edema. Although different proportions of the two types of negatively charged proteoglycan may account for the higher hydration levels in the posterior stroma compared to the anterior stroma (anterior cornea: 1.59 ratio of keratin sulfate to dermatan sulfate, 3.04 g H 2 O/g dry weight; posterior cornea: 2.23 ratio of keratin sulfate to dermatan sulfate, 3.85 g H 2 O/g dry weight), it appears that the directional orientation of the collagen fibrils and stromal lamellae probably have the greatest influence on how much each region thickens, or swells, as a result of increased hydration levels. Because the collagenous architecture of the stroma (i.e., limbus-to-limbus directional orientation of collagen fibrils) highly resists circumferential expansion, only anterior–posterior expansion occurs in the human cornea, predominantly in the posterior direction. This latter fact occurs because extensive lamellar interweaving occurs in the anterior third of the corneal stroma, while weaker bridging filaments (i.e., type VI and fibril-associated collagens with interrupted terminals collagens) occur diffusely throughout the entire corneal stroma. Furthermore, this lamellar interweaving also explains why the anterior third of the cornea mildly swells, whereas the remaining corneal stroma can swell to up three times its normal thickness. This anisotropic elasticity characteristic of the human cornea in swelling is important since the anterior corneal surface accounts for two-thirds of the refractive power of the eye. Because fibrotic corneal scars have random directionally oriented interweaving collagen fibrils, they have also been found to resist swelling under edematous conditions.

Therefore, although it is commonly stated that corneal thickness and interfibrillar spacing increase in a linear fashion to the hydration level of the corneal stroma, one needs to be aware that this relationship mainly applies to the mid and posterior stromal regions.
Etiology
Corneal edema is usually caused by one of two pathogenic mechanisms: endothelial cell dysfunction or high intraocular pressure (IOP). Common causes of endothelial dysfunction include Fuchs’ endothelial dystrophy, pseudophakic bullous keratopathy (i.e., from cataract surgery), trauma, other ophthalmic surgery (corneal transplantation, trabeculectomy/tube shunt glaucoma surgery), infections, and toxic anterior-segment surgery ( Box 9.4 ). Common causes of high IOP include uncontrolled glaucoma (acute angle closure glaucoma, neovascular glaucoma, pseudoexfoliation glaucoma), postoperative pressure spikes from retained viscoelastics, and medications (topical steroids).
Endothelial cell dysfunction
- •
Fuchs’ endothelial cell dystrophy
- •
Pseudophakic bullous keratopathy (cataract surgery)
- •
Trauma
- •
Other intraocular surgeries (glaucoma shunts, corneal transplantation)
- •
Infections (corneal ulcers or endophthalmitis)
- •
Toxic anterior-segment syndrome (toxic substances in anterior chamber)
High intraocular pressure
- •
Uncontrolled glaucoma (acute angle closure glaucoma, neovascular glaucoma, pseudoexfoliation glaucoma)
- •
Postoperative pressure spikes (retained viscoelastics)
- •
Medications (topical steroids)
Pathophysiology
Embryology to birth
During embryogenesis, the corneal endothelium forms during the 5th week of gestation as the first wave of neural crest-derived mesodermal cells form a two-cell layered primitive endothelium. By 8 weeks of gestation, a monolayer of cells is formed. The epithelium and endothelium remain closely opposed until 7 weeks of gestation (49 days) when a second wave of neural crest-derived mesodermal cells begins to grow centrally from the limbus between the epithelium and endothelium, producing the corneal stroma. By the 3rd month of gestation, Descemet’s membrane can be clearly recognized on histologic sections. Studies have inferred that during the 5th month of gestation the tight junctions completely form and the endothelial barrier is established; similarly, by 5–7 months’ gestation, the density of Na + /K + -ATPase pump sites eventually reaches adult levels so that the cornea becomes dehydrated and transparent. By the 7th month of gestation, the cornea resembles that of the adult in most structural characteristics other than size. At birth in the full-term infant, the horizontal diameter of the cornea is only around 9.8 mm (surface area 102 mm 2 ), or approximately 75–80% the size of an adult human cornea (note at birth, that the posterior segment is <50% the size of an adult human cornea).
Infancy to adulthood
The endothelium of a newborn infant cornea is composed of a single layer of approximately 500 000 neural crest-derived endothelial cells, each measuring around 5 µm in thickness by 20 µm in diameter, and covering a surface area of 250 µm 2 . The cells lie on the posterior surface of the cornea and form an irregular polygonal mosaic. The tangential appearance of each corneal endothelial cell is uniquely irregular, usually uniform in size to one another, and typically six-sided hexagons (which is the most energy-efficient and optimal shape to cover a surface area without leaving gaps). They abut one another in an interdigitating fashion with a 20 nm wide intercellular space between each other ( Figure 9.3A and B ). The intercellular space is known to contain discontinuous apical tight junctions ( Figure 9.3C ), or macula occludens tight junctions, and lateral gap junctions ( Figure 9.3D ). Thus, intercellular space of the corneal endothelium represents an incomplete diffusion barrier to small molecules. As corneal endothelial cells have numerous cytoplasmic organelles, particularly mitochondria, they have been studied and are presumed to have the second highest aerobic metabolic rate of all cells in the eye next to retinal photoreceptors. At birth, the central endothelial cell density of the human cornea is around 5000 cells/mm 2 . Because the corneal endothelium has very limited in vivo regenerative capacity (endothelial cells are currently hypothesized to proliferate at too low a rate in vivo to replace dying cells) and because aging results in progressive cellular senescence, particularly in the central regions of the cornea in part through the activity of the cyclin-dependent kinase inhibitor p21, there is a well-documented decline in central endothelial cell density with age that typically involves two phases: a rapid and slow component ( Figure 9.4 ). During infancy, the cornea continues to grow over the first 2 years of life, reaching adult size at 2 years of age with an average horizontal diameter of 11.7 mm (surface area 138 mm 2 ). Thereafter, it changes very little in size, shape, and optical properties. However, the only significant structure in the cornea that continues to grow after age 2 is the Descemet’s membrane as it gradually increases an additional 6–11 µm in thickness from birth to death. Due to corneal growth and age-related or developmentally selective cell death, during the fast component of cell loss, the central endothelial cell density decreases exponentially to about 3500 cells/mm 2 by age 5 and 3000 cells/mm 2 by age 14–20. Thereafter, a slow component of cell loss occurs where central endothelial cell density decreases to a linear steady rate between 0.3 and 0.6% per year, resulting in cell density measurements around 2500 cell/mm 2 in late adulthood. Because the corneal endothelium essentially maintains its continuity by migration and expansion of surviving cells, it is not surprising that the percentage of hexagonal cells decreases (pleomorphism) and the coefficient of variation of cell area increases (polymegathism) with age.
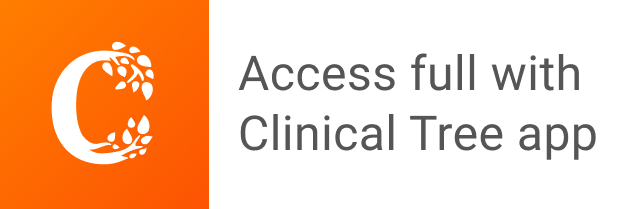