Fig. 4.1
Peripheral clocks and regulation of circadian physiology. The suprachiasmatic nucleus (SCN) is the major pacemaker of the circadian system that receives photic information directly from the retina and synchronizes peripheral oscillators found in other brain areas and peripheral tissues (entrainment). This is mediated by autonomic innervation, humoral signals, hormones, and the regulation of body temperature and feeding. Modified from Hickie et al., BMC Medicine, 2013, 11:79 with permission from BMC journals
Prior to describing the recent identification of clock systems in the auditory pathway, we will review general aspects of the structure and function of the mammalian circadian system.
4.3 Molecular Biology of Circadian Rhythms
4.3.1 Core Clock Genes
As evolution has progressed into more and more complex organisms, the biological clocks have incorporated more subtle mechanisms of regulation to incorporate the timing cues of a variety of zeitgebers and produce a variety of biological functions.
A major advance in the circadian field was the concept that biological rhythms are generated at the level of molecules that constitute autoregulatory feedback loops that self-regulate their transcription within a 24 h period. This process, which ballpark is conserved across various phylae, involves a series of activators that promote the transcription of repressors, which protein products translocate back in the nucleus to inhibit the transcription of the initial activators. The subsequent decrease of activator mRNA transcription reduces the level of inhibitory protein production, releasing the transcriptional machinery from its molecular breaks, thereby reinitiating the cycle.
In mammals, the circadian machinery has evolved into a complex cellular process to incorporate a large number of cues. The molecular clock can be paralleled to the machinery of normal clocks, with core large clocks regulating the rhythms of the smaller clocks. The molecular clock machinery is based on two interlocked autoregulatory transcriptional/translational feedback loops (TTFL) (Albrecht 2002; Kondratov et al. 2007; Lowrey and Takahashi 2004). In the center of the feedback loops, two basic helix-loop-helix (bHLH) transcription factors, CLOCK and BMAL1, dimerize and bind to E-box elements at the promoter regions of negative-feedback genes called Period (mPer1 and mPer2 in the mouse) and Cryptochrome (mCry1 and mCry2) to trigger their transcription. The CRY and PER protein products dimerize and form large corepressor complexes. As their concentration increases, they bind to CLOCK/BMAL1 complexes thereby interfering with its transcriptional regulation. The attenuation of their own transcription leads to a decrease in CRY and PER proteins. With a short life cycle, the decrease in PER-CRY complexes no longer interferes with CLOCK-BMAL1 heterodimers, and a new cycle of PER and CRY generation can follow (Fig. 4.2a).


Fig. 4.2
The molecular clock machinery. (a) Core loop: a complex of CBP/p300, CLOCK (C), and BMAL1 (B) binds to E-box on the promoter of Per and Cry genes (Per1, Per2, Cry1, and Cry2) to induce their transcription. Accumulation of PER/CRY complexes inhibit CLOCK and BMAL1 complexes, thereby repressing their own transcription. The progressive decline of PER and CRY proteins allows CLOCK and BMAL1 to initiate a new cycle of gene expression. Per1 and Per2 transcription can be additionally modulated by additional tran: heat shock factor (HSF) binding to heat shock elements (HSEs), cAMP-responsive element (CRE)-binding protein (CREB), and glucocorticoid receptor (GR) binding to glucocorticoid-responsive elements (GRE). (b) Interlocking loop: CLOCK and BMAL1 also trigger the expression of the orphan nuclear receptor genes Ror (Rorα, Rorβ, and Rorγ) and Rev -Erβ (Rev-Erβα and Rev -Erβ). The transcription of Bmal1 and Clock is regulated through competition between REV-ERβ repressors and ROR activators, acting on retinoid-related orphan receptor response elements (RORE). (c) Regulation of clock-controlled genes (CCGs): CLOCK and BMAL1 can regulate the transcription of CCGs by binding to E-box elements on their promoter area. These genes then are translated into CCG protein products and regulate physiological processes in a temporal way. Modified from Basinou et al., Hearing Research, 2016, in press with permission from Elsevier
An additional “interlocking” loop consists of ROR (RORα, RORβ, and RORγ) and REV-ERβ (REV-ERβα and REV-ERβ) proteins that are activated by the CLOCK/BMAL1 dimers. The resulting activated proteins recognize RORE response elements (RREs) within the promoter region of Bmal1 and Clock genes to regulate their transcription. RORs activate Bmal and Clock transcription, whereas REV-ERβs recruit the corepressor NCoR1 to inhibit gene transcription (Fig. 4.2b). Overall, the rhythmic regulation of Bmal1 transcription is thus typically in anti-phase with that of mPer, mCry, and mREV-ERβ mRNAs.
Disruption of both mPer genes, or both mCry genes, causes immediate behavioral arrhythmicity when the double knockout animals are placed in constant darkness (meaning in the absence of light entrainment), showing the essential role of PER and CRY in the maintenance of a functional clock (van der Horst et al. 1999; Zheng et al. 2001). Single mutants show continued clock oscillations indicating that there is partial compensation among family members.
Outside the core clock elements are found the clock-controlled genes, which output function is to control diverse physiological functions (Fig. 4.2c). The mechanism of regulation of clock-controlled genes resembles a lot to that of the core clock as suggested by the identification of main regulatory motifs of rhythmically expressed clock-controlled genes: E-boxes, D-boxes, and cAMP-responsive element (CREs) (Bozek et al. 2009, 2010; Korencic et al. 2014). For example in the liver, transcription factors of the bZIP family (DPB, TEF, and HLF) bind to D-boxes in the promoter area of the aldosterone receptor (CAR) gene, which in turn regulates the rhythms of detoxification via ALAS1 and cytochrome P450 expression (Gachon et al. 2006).
The accurate coordination of these transcriptional/translational feedback loops requires the tight control of posttranscriptional and posttranslational loops to generate a 24 h periodicity. Accumulating evidence shows that the activation and the stability of the core clock proteins is regulated by phosphorylation/dephosphorylation, SUMOylation, ubiquitination, acetylation/deacetylation, and polyADP-ribosylation (Dibner et al. 2010; Mehra et al. 2009). Even, it has been found that circadian rhythms of some specific metabolic functions such as peroxiredoxins in the absence of transcription (Edgar et al. 2012; O’Neill et al. 2011). At a broader scale, hormones, temperature, neurotransmitters, and second messengers (Dibner and Schibler 2015) also interfere with the expression of core clock genes such as Period, which allows resetting the phase of the core clock rhythms according to systemic cues.
At a broader scale, hormones, temperature, neurotransmitters, and second messengers also interfere with the expression of core clock genes.
It is interesting to note that near 10–20% of mRNA transcripts display circadian patterns of expression depending on the organ (Akhtar et al. 2002; Hughes et al. 2009; Panda et al. 2002; Storch et al. 2002; Ueda et al. 2002) but that 50% of rhythmic liver proteins are encoded by nonrhythmic mRNA transcripts (Mauvoisin et al. 2015), emphasizing the importance of translational and posttranslational modifications for the control of clock output pathways.
4.3.2 From Molecules to Physiology
A number of signals regulate the core clock and interfere with the rhythms of clock-controlled genes. This allows the cellular systems to respond in a timely manner to environmental changes with specific physiological outputs. As a consequence, cellular clocks appear ubiquitously present throughout the mammalian body (Yoo et al. 2004). Within a tissue, an ensemble of cellular clocks—although not all in synchrony—generate a coherent rhythmic physiological function. For all tissues to perform their functions on time, a master clock is needed to harmonize body rhythms. Located in the hypothalamus around the third ventricle, near 2000 neurons in rodents form the suprachiasmatic nucleus (SCN) that orchestrate the circadian system. The SCN was named the master clock after lesion and grafting studies demonstrated it is necessary and sufficient for the generation of body rhythms (Moore and Eichler 1972; Ralph et al. 1990; Stephan and Zucker 1972).
For all tissues to perform their functions on time, a master clock is needed to harmonize body rhythms.
Interactions between these neurons through chemical coupling and gap junctions facilitate their synchronization (Davidson and Menaker 2003), making the rhythms of the SCN robust enough to act as a pacemaker of all body rhythms. The SCN is entrained by light captured by the retina (Fig. 4.1), where intrinsically photoreceptive retinal ganglion cells (ipRGCs), which express melanopsin, project photic signals to the SCN via retinohypothalamic fibers (Hannibal and Fahrenkrug 2002; Hattar et al. 2002). This was evidenced in elegant genetic studies in mice, whereby the loss of these few hundred cells still allowed normal vision but not the synchronization of body rhythms to light input (Guler et al. 2008). Specific neurons (VIP) from the SCN transpose the electric signals to the cellular clock, through the activation of Ca2+-dependent kinase/CREB signaling cascades that initiate the cycles of molecular rhythms by triggering Per gene expression (Dibner et al. 2010). Neuronal interactions spread the rhythmic information across the SCN, which robust and synchronized output signals orchestrate central (e.g., olfactory bulbs and hippocampus) and peripheral tissues (e.g., liver, muscle, and adrenal glands) (Guilding and Piggins 2007; Richards and Gumz 2012).
The communication between the SCN and the other clocks occurs through autonomic innervation and to a second degree through the regulation of systemic cues such as body temperature, hormonal signaling, and feeding (Mohawk et al. 2012) (Fig. 4.1). In return, peripheral clocks provide feedback to the SCN regarding the internal status of the body by means of hormones and metabolites. A continuous and effective communication between external and internal signals allows to produce a coherent rhythmic physiological output.
Whereas the SCN is mainly entrained by light, peripheral clocks are regulated by signals either directly or indirectly controlled by the SCN. The SCN can directly influence peripheral rhythms thanks to the secretion of key factors such as prokineticin 2 (PK2) that can directly regulate locomotor activity rhythms (Cheng et al. 2002) and VIP that regulates core clock gene expression down to the liver and adrenal glands (Loh et al. 2011). Autonomic innervation plays an important role in the indirect communication between the SCN and peripheral clocks. For instance, the SCN projects efferent toward the paraventricular nucleus to control glucose homeostasis in the liver or glucocorticoid secretion by the adrenal glands (Ishida et al. 2005; Kalsbeek et al. 2004). Glucocorticoids (GCs) are of particular interest since they are powerful synchronizers of circadian rhythms. Glucocorticoid receptors are ubiquitously expressed nuclear receptors that recognize glucocorticoid response elements (GRE) present in the promoter and enhancer regions of core clock genes and clock-controlled genes. The glucocorticoid synthetic agent dexamethasone (DEX) is a well-known drug to synchronize circadian rhythms in culture.
Clocks from the heart, kidney, pancreas, lung, and thyroid glands are also controlled by autonomic nervous connections.
Rest and activity cycles drive feeding and body temperature rhythms that are additional zeitgebers. For instance, feeding cycles are important zeitgebers in the liver, the pancreas, the heart, and the kidneys (Dibner et al. 2010), possibly through glucose-sensing pathways and sirtuin signaling (Gachon et al. 2004a). Changes in body temperature (1–4 °C) can reset peripheral clocks via heat shock factor 1 (HSF1), which also regulates core clock gene expression through the binding to heat shock response elements (Reinke et al. 2008). However, it is thought that the robust rhythms from the SCN make it resistant to feeding and temperature changes (Abraham et al. 2010). Imposed feeding schedules in mice during resting phase can completely invert the circadian rhythms of peripheral tissues, while the SCN remains unaffected. These experiments also show that feeding cues can dominate hormonal signals (Abraham et al. 2010). Once normal feeding schemes are provided, the phase of the tissues resets in 2–3 days showing that the SCN can rapidly take over its role as a master clock. The importance of the SCN in synchronizing peripheral rhythms has been evidenced in lesion experiments where, in absence of the SCN, peripheral tissues became desynchronized with time (Yoo et al. 2004). It is now very well established that the SCN acts as a conductor of an orchestra, whereby peripheral tissues respond to the director’s instructions to provide a consistent physiological response.
4.3.2.1 The SCN and the Adrenal Glands: Teamwork for Body Synchrony?
If it can be concluded that almost any tissue harbors a circadian machinery, it remains that each organ harbors its own set of molecular elements to coordinate its physiological functions. In a study of Panda and others, the overlap between circadian transcripts in the SCN and the liver (among 650 oscillating transcripts) was compared, and only 28 transcripts were found common to the two tissues (Panda et al. 2002). Few of these 28 genes were core clock genes showing that the control of most circadian genes is highly tissue-specific, each peripheral clock being responsible for a specific output program dependent on the physiological functions.
The control of most circadian genes is highly tissue-specific. Each organ needs to tightly coordinate different functions at different times.
Each organ also needs to tightly coordinate different functions at different times. For instance, the liver is capable of regulating at appropriate times of the day gluconeogenesis and glycolysis, two chemically antagonistic processes. The temporal control of tissue- and time-specific physiological functions is done through the expression of clock-controlled genes that harbor various cis-regulatory elements to allow their on or off transcriptional states thereby generating a broad range of time-regulated cyclic activity within the same tissue.
An illustration of how clock-controlled genes are regulated by different zeitgebers has been shown in adrenalectomized mice, deprived of circulating glucocorticoids. Near 2/3 of rhythmic liver transcripts lost their rhythmicity in absence of adrenal glands, none of which were core clock genes (Oishi et al. 2005). These glucocorticoid-controlled genes were in a large part encoding liver enzymes (such as glucokinase, HMG-CoA reductase, and glucose-6-phosphatase). This proportion was replicated in a pharmacological study, in which DEX resynchronized 57% of liver genes from SCN-lesioned animals (Reddy et al. 2007) likely through a mechanism involving GR and CRY interactions (Lamia et al. 2011). Possibly, the SCN controls core clock rhythms, and secondary entrainment cures such as GCs orchestrate the rhythmicity of genes that regulate physiological functions in a given organ.
As such, the adrenal gland emerges as an important relay station downstream of the SCN to synchronize peripheral clock rhythms. Its endocrine functions are regulated in a circadian fashion (e.g., GCs show a rhythmic secretion pattern). The control of GC circadian secretion by the SCN is known from a long time and evidenced by SCN ablations (Moore and Eichler 1972; Stephan and Zucker 1972). In contrast, the pulsatile (or ultradian—see Chap. 2) pattern of GC secretion is independent of the SCN (Waite et al. 2012). The broad range of actions of GCs, from the regulation of stress or immune responses, as well as cognitive functions, suggests that the circadian actions of GCs might serve as key influencers of physiological rhythms. GCs exert their action via the glucocorticoid receptor (GR), expressed throughout the body including the cochlea (Meltser et al. 2014) and the brain—with the exception of the SCN (Balsalobre et al. 2000). GCs also activate the mineralocorticoid receptor (MR). The circadian secretion of GCs is a complex involvement of autonomic innervation controlled by the SCN, the hypothalamic-pituitary-adrenal (HPA) axis, and local adrenocortical clocks.
4.3.2.2 Physiological Functions Controlled by Circadian Rhythms and Their Dysregulation
Transcript analyses using qRT-PCR showed the circadian expression of core clock genes in the heart, lung, liver, stomach, spleen, and kidney (Yamamoto et al. 2004). Using transgenic rats driving luciferase expression under the control of Per1 promoter (Per1-luc), tissue explant cultures showed oscillations in the SCN, skeletal muscle, liver, lung, pineal, adrenal, and thyroid glands (Yamazaki et al. 2000, 2009). Another rodent model in which the luciferase-coding sequence was knocked-in the Per2 mouse locus (PER2::LUC) allowed to identify rhythmic oscillations in additional organs such as the cornea, the pituitary, and the retrochiasmatic area (RCA) (Yoo et al. 2004). How circadian cycles of luciferase expression are captured is illustrated in Fig. 4.3. As a consequence, numerous physiological functions manifest daily oscillations including detoxification processes by the liver, the kidney, and the small intestine (Gachon et al. 2006); carbohydrate and lipid metabolism by the liver, muscle, and adipose tissue (Lamia et al. 2008; Le Martelot et al. 2009); renal flow and urine production, blood pressure; and the rate of heart beats (Gachon et al. 2004a). Understanding of the physiological outputs from molecular clocks in each organ derives mainly from functional studies using mice lacking Bmal1, either systemically or in a tissue-specific manner, or mice lacking Per1 and/or Per2. For instance, mice lacking Bmal1 display a complete loss of circadian behavior, metabolic abnormalities, and subsequent reduced life span (Kondratov et al. 2006). Bmal1 or Clock mutants develop diabetes due to reduced insulin secretion by the pancreatic islets (Marcheva et al. 2010), a process specific to β-cells that regulates insulin secretion in a circadian manner (Perelis et al. 2015).


Fig. 4.3
Measures of circadian PER2::LUC oscillations in explant cultures. (a) The experimental system to analyze circadian gene expression in explants consists of isolating organs from mice expressing a luciferase fused to Period 2. (b) Organ explants are placed on membranes, and real-time bioluminescent imaging of PER2::LUC organs is collected using photomultiplier tubes which is highly sensitive and has low noise. Representative bioluminescence record of circadian PER2::LUC expression in cultured organs is then collected (c) and analyzed (d) for amplitude, period, and phase
Altered eating regimes, sleep and wake cycles, as well as medications can potentially alter the synchronicity of different organs and their harmonization at the body level leading to abnormal physiological functions.
Altered eating regimes, sleep and wake cycles, as well as medications can potentially alter the synchronicity of different organs and their harmonization at the body level leading to abnormal physiological functions. Shift workers suffer from the chronic desynchronization of their body with the regular environmental cues and of the SCN and peripheral clocks leading to increased prevalence of symptoms of the metabolic syndrome (Evans and Davidson 2013). Despite the fact that the SCN rapidly synchronizes its rhythms to light cues (e.g., in long-distance travelers changing time zones), their organs require more time to readjust their rhythms (jet lag). Underlying the human relevance, polymorphisms in hPER2 and hCRY2 associate with blood glucose levels. Those in hCLOCK are linked with obesity and hBMAL1 with hypertension and type 2 diabetes (Dibner and Schibler 2015).
4.3.3 Auditory System and Circadian Rhythms
Whether the auditory system harbors a molecular clock was unknown until recently. Several evidences pointed toward such circadian regulation. Firstly, noise can act as a zeitgeber to regulate body rhythms (Menaker and Eskin 1966; Reebs 1989). Secondly, outer hair cell function—measured by means of distortion products of otoacoustic emissions—appears to fluctuate throughout the day (Cacace et al. 1996; Haggerty et al. 1993). Thirdly, aminoglycoside-mediated ototoxicity is more damaging at night than during the day (Yonovitz and Fisch 1991). The later could however involve several mechanisms: different rates of liver detoxification around the clock could alter systemic bioavailability; the function of the cochlear blood barrier could fluctuate throughout the day making it more permeable to ototoxic drugs in the night than in the day; finally, the cochlear response to ototoxic insults could be weaker during the night due to varying metabolic rates throughout the day. Our laboratory revealed in an initial study that the cochlea possesses a robust molecular clock that responds differentially to day or night noise stimulation (Meltser et al. 2014). In a second work, we provided evidence that the inferior colliculus (IC), a midbrain relay of the auditory pathway, also has a molecular clock (Park et al. 2016). Here, we describe the findings of these two studies.
4.3.3.1 The Day-Night Susceptibility to Auditory Trauma
The fact that sensitivity to noise also varies at different times of the day was unknown until recently. Awake mice (non-anesthetized) exposed to a noise trauma (6–12 kHz, 1 h, 100 dB SPL) during the night (9 PM) displayed permanent shifts in hearing thresholds measured by auditory brainstem responses (ABRs) 2 weeks after noise trauma, whereas those exposed during daytime (9 AM) recovered to normal hearing thresholds (Meltser et al. 2014). Interestingly, ABRs measured 24 h post-trauma revealed equivalent shifts in hearing thresholds (15–30 dB, from 8 to 24 kHz) in day or night exposed animals. Although distortion products of otoacoustic emissions (DPOAEs) were not performed in this study, cochleograms revealed that no hair cell loss had occurred in both day and night noise groups. These findings suggest that immediate synaptic uncoupling, mainly caused by glutamate excitotoxicity after noise exposure, is similar during the day or during the night. Although levels of glutamate in the cochlea were not measured after day or night noise exposure, no differences were found in the wave I amplitude of the ABR 24 h post-trauma suggesting that animals were exposed to similar levels of sound in this paradigm (unpublished observations). This notion is important since nocturnal animals are more active during the night, whereas they are sleepy during daytime, and the resulting differences in hearing thresholds after day or night noise exposure could be due to varying levels of sound reaching the ear simply because of different behavioral patterns. This potential bias appeared to be negligible since fine and gross locomotor activity (measured by infrared beam breakage) showed no difference during day or night noise exposure (Park et al. 2016).
4.3.3.2 Involvement of Neurotrophic Signaling in the Differential Sensitivity to Noise Trauma Throughout the Day
A potential mechanism to explain the day and night differences in response to noise trauma included neurotrophic signaling in the cochlea (Meltser et al. 2014). Neurotrophins are important regulators of synaptogenesis and synaptic plasticity in the cochlea. Two important neutrophins, namely, neurotrophin-3 (NT-3) and brain-derived neurotrophic factor (BDNF), play an important role during cochlear development and in adult auditory recovery. Mice lacking NT-3 or BDNF, or their respective ligand-specific receptors of tropomyosin receptor kinase (TrkC or TrkB), lack a portion of the auditory neurons (Fritzsch et al. 2004). TrkC appears more important to auditory neuron development since its genetic ablation leads to the loss of 51–66% of auditory neurons, whereas loss of TrkB function causes a loss of only 15–20% of auditory neurons (from the high-frequency region). Their role appear inverted in the vestibular system where TrkB −/− mice show a loss of 56–85% of vestibular neurons whereas TrkC −/− only have 16–29% loss (Fritzsch et al. 2004). The similitude between the neurotrophin mutants and the receptor mutants provides strong evidence of their important contribution for the innervation in the inner ear.
The study from Meltser et al. revealed that the response of Bdnf transcription differed after day or night noise exposure. During the day, noise caused a 32-fold increase in Bdnf mRNA transcript levels, whereas no increase was after night noise. These findings indicate that the incapability of the cochlea to trigger a BDNF-dependent protective response after night noise could underlie the increased vulnerability to night noise exposure. Treatment before night noise exposure with dihydroxyflavone (DHF), a selective agonist of TrkB (Jang et al. 2010), restored the recovery of hearing thresholds to a level comparable to day noise exposure (in absence of drug treatment). Interestingly, night noise exposure caused a twofold reduction of the synaptic ribbons 2 weeks after noise exposure and DHF pretreatment protected synaptic ribbons (Meltser et al. 2014). These findings provide an evidence of the involvement of neurotrophins in the circadian recovery to noise trauma.
These results are somewhat conflicting with genetic studies that evaluated the contribution of NT-3 and BDNF to noise injury. In elegant genetic gain and loss of function studies, mice overexpressing Ntf3 or Bdnf via a tamoxifen-inducible Cre system (Ntf3 STOP :Plp1/CreER T or Bdnf STOP :Plp1/CreER T ) showed different responses to noise trauma, whereby only mice overexpressing Ntf3 showed accelerated recovery—not those overexpressing Bdnf (Wan et al. 2014). A potential explanation for the differences between Meltser et al. and Wan et al. is that DHF treatment (that mimics Bdnf actions on TrkB) was acute (single injection) and performed at night, whereas the genetic model of Ntf3 overexpression is comparable to a constitutively active (chronic) TrkC system. The role of Ntf3 in the differential responses to day or night noise exposure remains to be investigated.
4.3.3.3 Molecular Biology of Cochlear Circadian Rhythm
The differential response to day or night noise trauma led to the hypothesis that the cochlea could harbor a clock machinery. Using a mouse reporter in which the luciferase gene was fused in frame with the endogenous Period 2 gene (PER2::LUC) (Yoo et al. 2004), real-time bioluminescence from cochlear explants could be monitored as a readout of PER2 expression. PER2::LUC rhythms from the cochlea ex vivo appeared as ample as those from the liver (Fig. 4.4a), and after fading out, these rhythms could be kicked off with dexamethasone treatment, a known synchronizing agent (Meltser et al. 2014). PER2 protein expression originated from hair cells and spiral ganglion neurons (Fig. 4.4b).


Fig. 4.4
Circadian oscillations in the cochlea: representative bioluminescence records of circadian PER2::LUC expression in cultured adult cochleae explants (a). (b) Immunostaining of PER2 in a cochlea of intact adult CBA/CaJ mouse shows the localization of the protein in inner and outer hair and supporting cells of the organ of Corti and in the spiral ganglion neurons of the cochlea. Scale bar: 50 mm. (c) Temporal expression of Per1, Per2, Rev -Erβα, and Bmal1 mRNAs in the cochlea assessed by q-RTPCR. The vertical axis shows normalized mean values ± SEM (n = 3–4). The horizontal axis shows the sampling circadian time (CT) across 24 h at which the animals were sacrificed and samples collected. The shaded area illustrates the dark phase of the day from CT 12 to CT 0. All conditions were plotted as relative percentage change using CT 0 as baseline value. Basinou et al (2017)
The expression of core clock genes was further evidenced by qRT-PCR, thanks to improvements in a method of cochlear RNA extraction, yielding high quantities of RNA of a quality suitable for such molecular analyses (Vikhe Patil et al. 2015). Subsequently, the circadian expression of Per1, Per2, Bmal1, and Rev -Erβα was revealed, demonstrating that the cochlea harbors key components of the core clock machinery (Fig. 4.4c). Presence of additional circadian genes was revealed using a more sensitive methodology, namely, the NanoString nCounter (Cederroth and Canlon, unpublished observations).
The cochlea harbors key components of the core clock machinery.
Interestingly, noise exposure at night affected to a greater extent than day noise exposure the amplitude of Per1, Per2, Bmal1, and Rev -Erβα mRNA expression, a finding further validated via the monitoring of PER2::LUC rhythms in vitro. In addition, activation of TrkB with DHF modulated PER2::LUC oscillations to a greater extend in the day than in the night (Meltser et al. 2014). How these changes in cochlear rhythms are coupled to the physiological responses to noise trauma is a challenging area of research, but overall these findings illustrate the complex and interdependent links between noise, neurotrophins, and circadian rhythms in the cochlea.
4.3.3.4 Circadian Rhythms in the Inferior Colliculus
Recent experimental work from our laboratory revealed that the IC, an important relay of the auditory pathway involved in tinnitus, also possesses a molecular clock (Park et al. 2016). PER2::LUC rhythms in vitro were also captured in whole mount or sectioned IC (Fig. 4.5a). Immunohistochemistry revealed that PER2 is homogeneously expressed throughout the IC according to the different subdivisions, namely, the central nucleus of the IC (CIC), dorsal cortex of the IC (DIC), and external cortex of the IC (ECIC) along the rostro-caudal axis (Fig. 4.5b). NanoString nCounter arrays revealed circadian mRNA transcript profiles for Per1, Per2, and Bmal1, among others, and the clock-dependent genes Dbp (Fig. 4.5c). Curiously, the analysis of PER2::LUC rhythms indicates that the IC in the postnatal stage showed more robust circadian oscillations than the adult stage. In postnatal day 4 (P4) ICs, 100% of cultured IC oscillated, and in adult ones (P50), this dropped to 30%. To explain this phenomenon, multiple factors were investigated such as the experimenter, the gender of the animals, whole mount vs sections, the thickness of the sections, and their orientations (coronal, sagittal, horizontal), but none explained this decline in successful oscillations. A potential explanation is that aging influences the synchronicity of the oscillations, which suggests that a greater proportion of ICs might fail to show oscillations in adult stage, or that as an animal ages, components of the expression of core clock machinery could decrease until affecting the whole machinery.
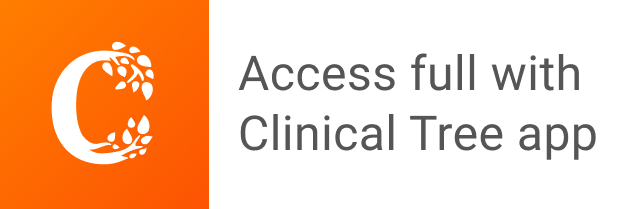