Fig. 22.1
The Ocular Response Analyzer (ORA) (Reichert, Inc. Depew, New York, USA)

Fig. 22.2
The CorVis ST (Oculus Optikgerate GmbH, Wetzlar, Germany)
Both instruments work in a similar manner and are by analyzing the deformation of the cornea an air pulse. The ORA system provides two biomechanical parameters, the corneal hysteresis and corneal resistant factor, which have shown to be reproducible in normal patients and be altered in eyes with corneal ectatic disorders [9]. On the other hand, the CorVis utilizes a Scheimpflug camera which is able to assess more than 4000 frames per second aiming to evaluate the dynamic behavior of the cornea during the deformation process [10].
Corneal biomechanical behavior can also be evaluated by using ex vivo techniques . One of the most widespread procedures used for that purpose is by means of the analysis of the modulus of elasticity of the corneal tissue. For this technique, stress–strain measurements are determined performing extensiometry experiments. In this type of experiments, a strip of corneal tissue is clamped into a mechanical press which pulls the ends of the corneal strip. Then, by means of strain control, samples undergo deformation in a ramp with a constant speed. The equipment registers the force that is necessary to perform this deformation and the stress–strain graphics are plotted. This way, the elastic modulus of the sample can be determined and denotes the relationship between the stress and the strain of the sample. Corneas with a higher elastic modulus are supposed to be stiffer and able to bear a bigger amount of load in comparison to those with a lower elastic modulus and therefore more weak from the biomechanical point of view.
22.3 Carbon Nanomaterials: Structure and Properties
Nanomaterials have emerged in the last two decades as highly versatile platforms with unparalleled properties compared to systems with the same composition in the bulk phase. The excellent properties of materials are based in their precise engineering at the nanometer length scale (ultra-small size, large surface area-to-mass ratio, and high reactivity), thus providing exciting properties not only in traditional fields such as engineering and electronics, but also in new research branches such as therapeutics (e.g., drug delivery, cancer treatment), tissue engineering, and so on. These carefully designed nanoscale agents provide more efficient systems in applications such as drug delivery , with controlled release, lower therapeutic toxicity, and reduced healthcare costs. Taking into account the vast scope of materials for biomedicine (polymers, nanoparticles, etc.), in this section we will briefly focus on carbon nanostructures and their biomedical application.
Although activated carbon materials (activated charcoal) have been widely used in medicine since many decades (for instance, to reduce intestinal gas, treat poisoning, lower cholesterol levels, etc.), it was not until the 90s, when a large revolution started thanks to the discovery of new fibrous carbon nanostructures (e.g., mainly carbon nanotubes and carbon nanofibers), followed by the isolation of a single graphene in 2010. These carbon materials together with graphene oxide nanoribbons and nanodiamonds have made a significant impact in science and technology, from enhancing the mechanical properties of composite materials, to miniaturizing electronics, providing more efficient energy storage and facilitating the early detection and treatment of diseases [11–16]. It is the versatility of the carbon nanostructures that makes them attractive and versatile for various applications. They are nanoscopic in size, have high length-to-width and surface-to-volume ratio, can have hollow core and are chemically inert to many reagents but can be easily modified to possess different surface chemistry. Among them, carbon nanotubes (CNTs) , discovered by Japanese scientist Iijima in 1991, have being postulated as the most attractive carbon nanomaterials due to their intrinsic properties such as impressive structural, mechanical, and electronic properties (they have conducting or semiconducting properties) and their unique optical properties [14–16]. These nanomaterials are allotropes of carbon, made of graphene, and they can be described as a rolled up graphene sheet with sp2 carbons giving rise to cylindrical tubes with nanometer scale in diameter and several millimeters in length [17, 18]. Depending on the number of cylindrical graphene layers, CNTs can be classified as single-wall carbon nanotubes (SWCNTs ) or multiwalled carbon nanotubes (MWCNTs). SWCNTs consist of a single graphene cylinder with diameter between 0.4 and 2 nm, whereas MWCNTs consist of two to several coaxial cylinders, with an outer diameter ranging from 2 to 100 nm.
Figure 22.3 shows TEM images and schematic drawing of carbon nanotubes : single-walled carbon nanotube (SWCNT ) and a multiwalled nanotube (MWCNT ) [14].

Fig. 22.3
TEM images and schematic drawing of carbon nanotubes: single-walled carbon nanotube (SWCNT) and a multiwalled nanotube (MWCNT). Reprinted from S. Iijima, “Carbon nanotubes: past, present and future”, Physica B 323 (2002) 1–5 [14], with permission of Elsevier
CNTs exhibit high tensile strength, stiffness, and ductility [19]. In fact, the tensile strength of SWCNTs is 100 times that of steel, making them the strongest material ever known [16]. Concerning the electrical properties , SWCNTs can be metallic or semiconducting depending on their helicity, whereas MWCNTs exhibit a range of electronic behavior (metallic, semiconducting, and semimetallic) [20]. However, the reactivity of carbon surfaces varies greatly with surface microstructure, cleanliness, and functional groups [21].
As described earlier, although carbon nanotubes were first used as additives to improve the performance of structural materials for electronics, optics, plastics, etc., since the beginning of the twenty-first century they have been introduced in pharmacy and medicine for drug delivery systems in therapeutics. Nowadays they are used as excellent drug vehicles for drug delivery by penetration into the cells while keeping the drug intact without metabolism during transport in the body [15–18]. When bonded to CNTs, these drugs are delivered more effectively and safely into cells as compared to other conventional methods. CNTs have also being applied to bind antineoplastic and antibiotic drugs for cancer and infection treatments, respectively. Similar linkages of biomolecules (genes, proteins, DNA, antibodies, vaccines, biosensors, cells, etc.) have also been assayed for gene therapy, immunotherapy, tissue regeneration, and diagnosis of different ailments [22–24]. Therefore, the applications of CNTs are very wide and very exciting in a wide variety of disciplines.
CNTs of various diameters, lengths, and structure can be fabricated using a variety of methods from arc discharge method (using arc vaporization of two carbon rods), laser ablation method (using graphite), catalytic chemical vapor deposition (C-CVD), using hydrocarbon sources such as CO, methane, ethylene, etc., plasma enhanced chemical vapor deposition (C-PECVD), and template-based CVD. After preparation, CNTs usually contain amorphous carbon, fullerenes, and catalytic metal particles (e.g., Co or Ni) as impurities that must be carefully removed before any further processing. Usually the purification process involves several steps from an initial oxidation upon heating to ~350 °C in air, to remove amorphous carbon, followed by a nonoxidizing acid refluxing to remove the metal nanoparticles and a final annealing at temperatures in excess of 1000 °C in vacuum to remove all the defects created in early steps [25].
22.4 Carbon Nanomaterials in Biomedical Applications
One of the most important concerns after the preparation of the purified carbon nanomaterials and before their application in any biomedical application concerns their functionalization . The introduction of surface functional groups in the open or defect carbon sites (principally oxygen surface groups) allows increasing their solubility in aqueous solutions, and in addition improves the biocompatibility and reduces the toxicity for their medical application [26]. The incorporation of functional groups can be performed by covalent attachment (e.g., incorporation of carboxyl (-COOH) groups to the carbon atoms at edges or defect sites of the nanotubes via an oxidation treatment with strong acids), or by noncovalent attachment (e.g., physisorption of large macromolecules via noncovalent interactions with the aromatic surface of the nanotubes), depending on the final application. Upon functionalization, carbon nanotubes become more hydrophilic and consequently, their solubility in physiological media is highly improved. Furthermore, the presence of functional groups improves the anchoring of drugs and biomolecules (e.g., enzymes, biosensors, proteins, DNA, etc.).
Figure 22.4 shows the chemical modification of carbon nanotubes through thermal oxidation using strong acids, followed by a subsequent esterification or amidization of the carboxyl groups [27].

Fig. 22.4
Chemical modification of carbon nanotubes through thermal oxidation using strong acids, followed by a subsequent esterification or amidization of the carboxyl groups. Reprinted from K. Balasubramanian, M. Burghard, “Chemically functionalized carbon nanotubes”, Small, 1, 180–192 (2005) [27], with permission of John Wiley and Sons
The main applications of carbon nanomaterials in medicine and pharmacy are based on their unique structural, electronic, and mechanical properties. These applications include electrochemical sensors, biosensors, drug deliver to organs or cells, vaccine vehicles, tissue engineering, etc.
Due to the high ratio of surface area to volume, CNT-based electrodes exhibit a significant increase in the signal-to-noise ratio compared to other electrochemical sensors with the corresponding increase in the detection sensitivity. For instance, Huang et al. used ferrocene functionalized SWCNTs noncovalent film to detect l-glutamate [28]. The unique interdigitated structure of the ferrocene/SWCNT film provided a high catalytic efficiency, a high sensitivity, and a fast response during the detection at low concentrations. Similar electrochemical sensors were developed by Lin et al. for selective detection of glucose. Glucose oxidase was covalently immobilized on CNTs surface through the EDC/sulfo-NHS linker moieties [29]. Experimental results show that the biosensor effectively performs a selective electrochemical analysis of glucose in the presence of common interferents (e.g., acetaminophen, uric and ascorbic acid).
SWCNT and MWCNT-based field effect transistors can also be used as sensors for detection of gases and vapors with high sensitivity based on the variations in their electrical resistance with changes in the environment (e.g., when molecules adsorb on the surface). Compared to other solid-state sensors at room temperature, CNTs sensors exhibit a faster response and a substantially higher sensitivity. These studies include detection of small molecules such as nitrogen dioxide, ammonia, and oxygen, as well as larger odor molecules such as trimethylamine, dinitrotoluene, and dimethylphosphonate [30, 31]. The application of CNTs as a sensor has been extended to larger macromolecules. For instance, researchers of the NASA Ames research center have used CNTs to detect ribonucleic acid (RNA), DNA, and DNA polymerase chain reaction (PCR) amplicons in the solution [32, 33].
Another application of CNTs concerns its use in bone regeneration . Mwenifumbo et al. evaluated the growing of human osteoblastic cells (SaOs-2) on MWCNT constructs and compared to a control based on flat highly ordered pyrolytic graphite (HOPG) surfaces to investigate cellular attachment, cell metabolic activity, and construct cytocompatibility [34]. Experimental results demonstrate that cells attach and survive on the MWCNT constructs, with higher metabolic activity compared to control surfaces and metabolic activity negatively correlating with nanotube diameter. Usui et al. showed that MWCNTs are highly biocompatible [35]. Furthermore, MWCNTs inhibit osteoclastic bone resorption in vivo, inhibit osteoclastic differentiation, and suppress the expression of a transcription factor essential for osteoclastogenesis in vitro. CNTs have also being investigated for other tissue types. Hanui et al. evaluated the effect of MWCNTs on IMR-32 human neuroblastoma cells [36]. MWCNTs only bound to the cells with low cytotoxicity, high biocompatibility, and with a scarce effect on the nerve system.
In terms of safety, in vitro tests (following the appearance of alkaline phosphatase activity) and in vivo tests (by implantation of MWCNT/CHI scaffolds adsorbed with rhBMP-2 in muscle tissue and evaluation of the ectopic formation of bone tissue) show a high biocompatibility, the composite being biodegradable. However, experimental results have anticipated that the incorporation of the CNTs has no clear beneficial effects compared to the base biomaterials, whereas the safety remains an important drawback. Due to the biopersistent character of CNTs and their accumulation in the cells, the CNTs excretion or biodegradation after absorption in the body remains a safety issue before scaffold biomaterials can be applied in human bodies. In despite of this result, other authors that have also analyzed the biocompatibility of such nanomaterials have demonstrated their safety. In the work performed by Zhao et al. the authors observed that CNTs did not interfere with metabolic activity of the cell types that were under study [37]. In the same line, low toxicity and inert biological response have also been reported in another study that investigated the behavior of such nanomaterials in peripheral blood of experimental animals [38]. Nowadays, there are other several investigators that have reported in the scientific literature that carbon nanomaterials are biologically compatible, they are not toxic for the tissues, and that also cleared from circulation when injected intravenously [39–43].
22.5 Carbon Nanomaterials and Biomechanics
In the field of biomedicine , CNTs have also being applied as reinforcement materials for scaffolds in tissue regeneration, i.e., as a reinforce for weak points of existing scaffolds. For instance, incorporation of CNTs to a collagen scaffold has demonstrated an improved mechanical behavior because of the favorable properties of CNTs [44, 45]. Zhang et al. reported that a composite of poly(l-lactide) and MWCNT showed increased direct current conductivity, crystallization, plasticization of the polymer matrix, and growth inhibition of fibroblast cells [46]. Abarrategi et al. fabricated MWCNT-chitosan (CHI) scaffolds composed of MWCNT (up to 89 wt%) and with a well-defined microchannel porous structure as biocompatible and biodegradable supports for culture growth [47]. Other authors have also shown that CNTs may lead to an improvement in the mechanical properties of the collagen. In the work conducted by Cao et al., the authors found an increase of the static tensile modulus and strength of the collagen when adding CNTs to their samples [44]. In the same line of investigation, Tosun also observed a significant enhancement in the rigidity of the collagen gels treated with CNTs [48].
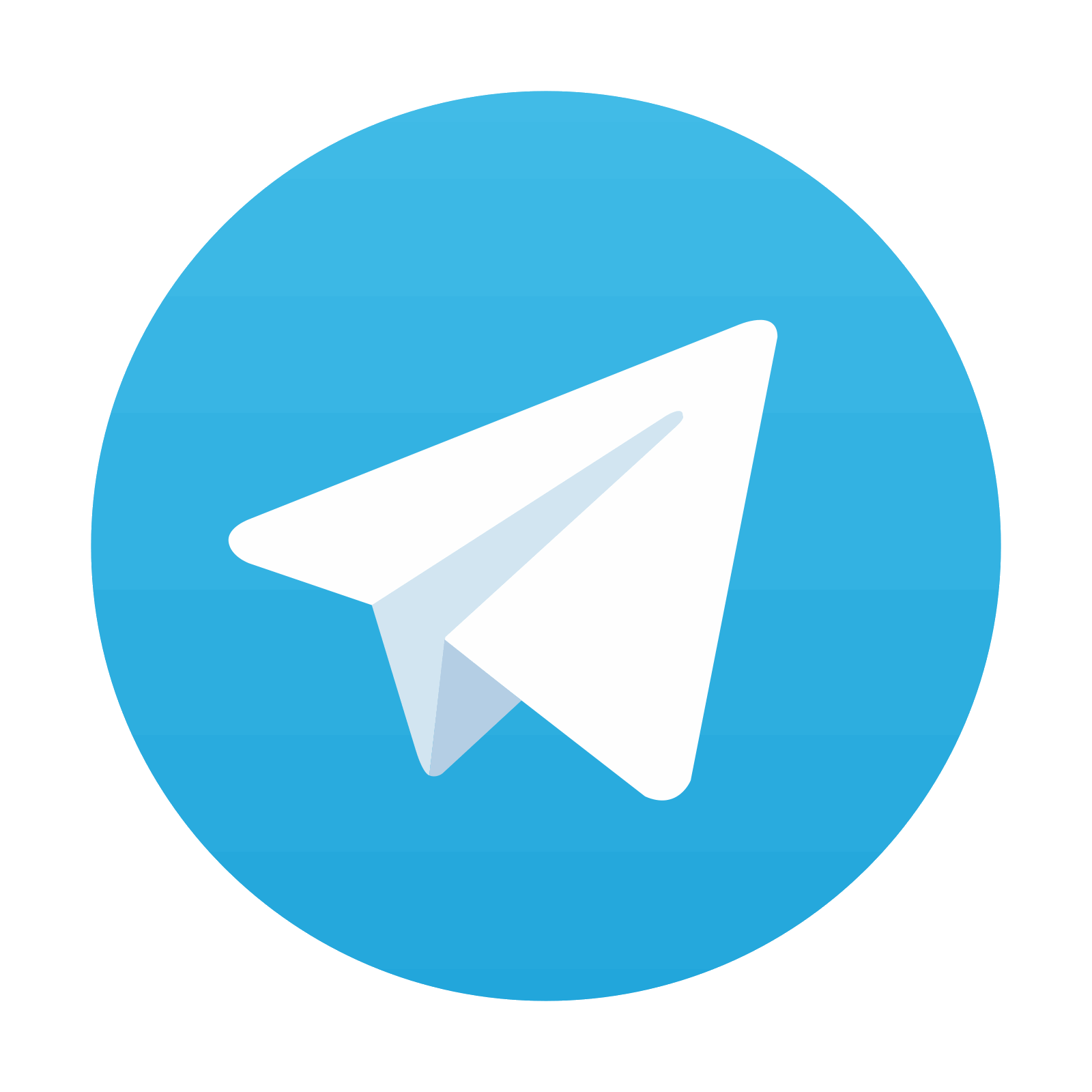
Stay updated, free articles. Join our Telegram channel
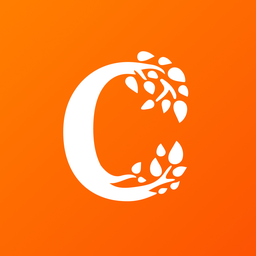
Full access? Get Clinical Tree
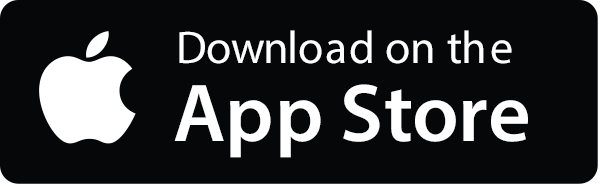
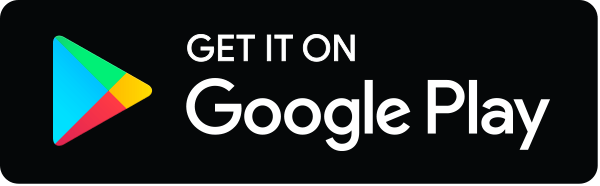