Purpose
To provide a critical review of the relationships between blood pressure, ocular blood flow, and glaucoma and the potential for glaucoma treatment through modulation of ocular perfusion.
Design
Summaries of the pertinent literature and input from glaucoma researchers and specialists with relevant experience.
Methods
Review and interpretation of selected literature and the results of a 1-day group discussion involving glaucoma researchers and specialists with expertise in epidemiology, blood flow measurements, and cardiovascular physiology.
Results
Accurate, reproducible, and clinically relevant measurements of blood flow within the optic nerve head and associated capillary beds are not fully achievable with current methodology. Autoregulation of blood flow in the retina and optic nerve head occurs over a large range of intraocular pressures and blood pressures. Regulation of choroidal blood flow is provided by a mix of neurohumoral and local mechanisms. Vascular factors may be important in a subgroup of patients with primary open-angle glaucoma, and particularly in patients with normal-tension glaucoma and evidence of vasospasm. Low ocular perfusion pressure and low blood pressure are associated with an increased risk of glaucoma in population-based studies. The physiologic nocturnal dip in blood pressure is protective against systemic end-organ damage, but its effects on glaucoma are not well elaborated or understood. Large-scale longitudinal studies would be required to evaluate the risk of glaucomatous progression in non-dippers, dippers, and extreme nocturnal blood pressure dippers.
Conclusions
Decreases in perfusion pressure and blood pressure have been associated with glaucoma. However, there is no evidence to support the value of increasing a patient’s blood pressure as therapy for glaucoma. Such recommendations are not currently warranted, since we lack crucial information about the microvascular beds in which perfusion is important in glaucoma, and the appropriate methods to evaluate their blood flow. There are also cardiovascular safety concerns associated with treatments designed to increase ocular perfusion pressure and blood flow by increasing blood pressure, especially in elderly patients. For these reasons and with present evidence it is unlikely that safe and effective glaucoma treatments based on altering optic nerve perfusion will soon be available.
Glaucoma is a family of optic neuropathies having in common a characteristic cupping of the optic nerve head and a distinctive pattern of visual field loss for which increased intraocular pressure (IOP) is an important risk factor. From the time of its earliest recognition, there has been a debate about its etiology and preferred treatment. Elevated IOP has long been thought to increase the risk of glaucoma by causing abnormalities of the optic nerve head at the level of the lamina cribrosa, affecting the intracellular transport within the retinal ganglion cell axons or by causing vascular abnormalities that lead to ischemic damage. When von Graefe introduced iridectomy as a successful treatment for acute glaucoma in the mid-1800s, he postulated that it was helpful because it reduced aqueous production and IOP, while Jaeger believed that it was helpful because it altered ocular blood flow to improve nutrition and decrease inflammation.
The role of IOP as a risk factor for glaucoma is now well established, and current treatment of glaucoma aims to reduce IOP to a target pressure low enough to prevent or significantly slow progression. The role of optic nerve and retinal blood flow and ischemia in glaucoma is not clear. In the 1990s, Hayreh, Drance, and others raised the important issues of systemic hypotension and nocturnal blood pressure dips in the progression of glaucoma and the desirability of accurate clinical measurements of ocular blood flow. Interest in these topics has resurged in light of recent epidemiologic data concerning low blood pressure and low calculated “ocular perfusion pressure” as risk factors for the development and progression of glaucoma. The purpose of this Perspective is to critically evaluate the current evidence for reduced blood flow in the pathology of glaucoma, its measurement, and its iatrogenic perturbation as a means of treatment for glaucoma.
Anatomy and Clinical Measurements of Ocular Blood Flow
The ocular circulation is composed of a complex arterial supply and an even more complex venous drainage system. The ophthalmic artery and its tributaries, the posterior ciliary arteries and the central retinal artery, provide arterial blood flow to the posterior segment. The short posterior ciliary arteries from the choroid mainly supply the prelaminar portion of the optic nerve, with a minor contribution to the surface of the disc from fine branches of the central retinal artery.
A variety of techniques have been used to measure ocular blood flow. Fluorescein angiography can be used to estimate the arteriovenous transit time, but such estimates do not represent a robust measure of regional blood flow. The perfusion of microvascular beds can be evaluated qualitatively with this technique.
The microsphere technique has been used since the early 1970s to measure ocular blood flow in animals. The advent of fluorescent microspheres simplified the technique, and recent refinement of the optimal dose and size of microspheres may provide more accurate estimates of regional optic nerve head blood flow. The technique is limited by the need to euthanize the animal to harvest the tissues and provides a limited number of “snapshot” measurements.
Laser Doppler velocimetry (LDV) determines the velocity of blood cells in the larger retinal blood vessels. Volumetric blood flow to or from the retinal area served by the artery or vein can be calculated if the velocity is combined with measurements of vessel diameter at the same site. LDV cannot be used to measure optic nerve head blood flow.
Laser Doppler flowmetry (LDF) or laser speckle flowgraphy (LSFG) measures flux (mean velocity times the number of moving cells) based on the Doppler shift imparted to light scattered by blood cells moving in the microcirculation of a laser-illuminated tissue. Both techniques are used to measure microcirculatory perfusion in the optic nerve head in animals and humans. The techniques require skill and ideal subjects (eg, clear media and perfect fixation). They cannot be used to measure flow in large vessels, and neither provides measurements in absolute units. Moreover, the depth to which LDF and LSFG measurements penetrate the optic nerve head is still being resolved and probably varies with differences in tissue light scattering elements between subjects (eg, a normal versus a cupped disk). Consequently, there is some uncertainty regarding which vascular beds are actually being measured and whether those are the vascular beds relevant in glaucoma.
Color Doppler imaging (CDI) evaluates erythrocyte velocity in the large ophthalmic vessels (the ophthalmic artery, central retinal artery, and short posterior ciliary arteries). CDI provides the peak systolic velocity (PSV), the end diastolic velocity (EDV), and the mean velocity. The resistive index ([PSV-EDV]/PSV) is often calculated, but for the central retinal artery, it correlates poorly with direct measures of retinal vascular resistance. Velocity measurements in the ophthalmic artery are also difficult to interpret since much of blood flow in the ophthalmic artery does not perfuse the eye, so velocity does not change when IOP is raised as high as 45 mm Hg. Several studies have suggested that erythrocyte velocity measured with color Doppler imaging may predict the risk of glaucoma progression, but such findings among various studies have not been in the same vessels or for the same measures. The large variation in measurements among individuals, probably attributable in part to differences in tissue density and vascular organization, complicates the interpretation of color Doppler imaging. The measurements are also highly variable and dependent on probe placement. There is evidence that erythrocyte velocity measurements may underestimate blood flow, and instantaneous measurements may not provide an accurate estimation of either flow or change in flow over longer, more relevant periods of time.
The limitations of these techniques have made it difficult to determine the fundamental characteristics of ocular blood flow in glaucoma and in normal controls. The measurements in humans are in general quite noisy, or variable. There is currently no widely accepted consensus regarding which techniques should be used to evaluate blood flow or how the results should be interpreted. None of the methods used to estimate blood flow have been standardized or externally validated for humans. Ocular blood flow measurements are not currently used in the diagnosis or management of patients with glaucoma.
Physiology and Autoregulation of Ocular Blood Flow
The driving force for ocular blood flow is the ocular perfusion pressure (OPP), defined as the ocular arterial pressure minus the IOP. A relationship between OPP and ocular blood flow has been demonstrated in several animal models, where arterial pressure can be precisely controlled. In this model, choroidal blood flow ceases when the OPP is zero, indicating the ear artery pressure is a reasonable estimate of the arterial pressure entering the choroid. However, in humans and other species lacking an artery for surrogate measurement, arterial pressure is measured at a distant site (eg, the brachial artery) and corrected for the hydrostatic column effect to obtain an estimated ophthalmic artery pressure. Ophthalmodynametric measurements in humans of the IOP at which retinal blood flow ceases indicate this is reasonable for the central retinal artery. It should not be assumed that this holds for the arteries supplying the other ocular circulations. For example, rat ophthalmodynamometric measurements indicate that the pressure is approximately 10 mm Hg lower in the short posterior ciliary arteries than in the central retinal artery. Qualitatively similar results are reported for humans with fluorescein angiography during acute IOP elevation.
In clinical studies, brachial arterial pressure has been used as a surrogate for the ocular arterial pressure in the calculation of OPP. They are not equivalent because when an individual is sitting or standing, the ocular arterial blood pressure is lower than the brachial arterial pressure because of the effect of gravity (the hydrostatic column effect). Therefore, the OPP calculated with the uncorrected brachial artery blood pressure is not the actual physiologic OPP. Also, we cannot assume that the difference between the ocular and brachial arterial pressures is the same in normal and diseased vascular beds.
Blood flow is determined not only by OPP, but also by vascular tone (resistance). Regulation of blood flow may occur through changes in vascular resistance (vasoconstriction or vasodilation) independently of changes in the OPP. Although the physics of ocular blood flow are the same in nonprimate animal models and in humans, the regulation of ocular blood flow is likely to vary among species because of anatomic differences. For example, in contrast to humans, the rabbit retinal circulation is negligible and the retina is nourished mostly from the choroid. Other differences between animal models and humans, including postural changes from a 4-legged position to upright and differences in circadian activity, may also make it difficult to extrapolate findings concerning the regulation of blood flow from one species to another.
Autoregulation of ocular blood flow is designed to match the metabolic needs of ocular tissues to maintain consistent blood flow despite changes in the OPP. In the retinal circulation, blood flow autoregulation is desirable because retinal metabolic activity and the consequent need for oxygen, nutrients, and waste removal are blood flow dependent. Because the choroidal vascular bed is a high flow bed, with the smallest arteriovenous oxygen difference of any in the body, the extent to which metabolic autoregulation of choroidal blood flow occurs is not clear. However, measurements of the retinal PO 2 gradient in primates show that normal choroid blood flow provides adequate oxygen delivery to the photoreceptor inner segments under light-adapted conditions and marginal oxygen delivery under dark-adapted conditions. Thus, despite the low arteriovenous oxygen difference, the choroid is not over-perfused for retinal oxygen delivery, and even mild reductions in choroidal blood flow can render the photoreceptor inner segments hypoxic. Efficient autoregulation of choroidal blood flow over defined ranges of ocular arterial pressure and IOP has been observed in rabbits. In contrast, in cats and primates in which the radioactive microsphere technique was used to measure ocular blood flow, induction of ocular hypertension resulted in decreased blood flow through the choroid while retinal blood flow remained at normal levels, suggesting a marked difference in autoregulatory capacity between the choroidal and retinal vascular beds. A more recent primate study with laser speckle flowgraphy to measure blood flow demonstrated autoregulation of optic nerve head blood flow at some levels of blood pressure. Studies with laser Doppler flowmetry in humans also indicate optic nerve head autoregulation when IOP is increased stepwise with a suction cup. Moreover, the optic nerve head hyperemic response to flicker light stimulation in animals and humans indicates efficient autoregulatory neurovascular coupling to match metabolism and perfusion.
Autoregulation of both the retinal and choroidal blood flow may make it difficult to modify ocular circulation for therapeutic benefit. Moreover, there may be unforeseen adverse consequences. For example, in a rabbit model, administration of vasodilators to increase blood flow causes an increase in ocular blood volume, which leads to a large, transient spike in IOP that could damage an already diseased optic nerve.
Physiology and Autoregulation of Ocular Blood Flow
The driving force for ocular blood flow is the ocular perfusion pressure (OPP), defined as the ocular arterial pressure minus the IOP. A relationship between OPP and ocular blood flow has been demonstrated in several animal models, where arterial pressure can be precisely controlled. In this model, choroidal blood flow ceases when the OPP is zero, indicating the ear artery pressure is a reasonable estimate of the arterial pressure entering the choroid. However, in humans and other species lacking an artery for surrogate measurement, arterial pressure is measured at a distant site (eg, the brachial artery) and corrected for the hydrostatic column effect to obtain an estimated ophthalmic artery pressure. Ophthalmodynametric measurements in humans of the IOP at which retinal blood flow ceases indicate this is reasonable for the central retinal artery. It should not be assumed that this holds for the arteries supplying the other ocular circulations. For example, rat ophthalmodynamometric measurements indicate that the pressure is approximately 10 mm Hg lower in the short posterior ciliary arteries than in the central retinal artery. Qualitatively similar results are reported for humans with fluorescein angiography during acute IOP elevation.
In clinical studies, brachial arterial pressure has been used as a surrogate for the ocular arterial pressure in the calculation of OPP. They are not equivalent because when an individual is sitting or standing, the ocular arterial blood pressure is lower than the brachial arterial pressure because of the effect of gravity (the hydrostatic column effect). Therefore, the OPP calculated with the uncorrected brachial artery blood pressure is not the actual physiologic OPP. Also, we cannot assume that the difference between the ocular and brachial arterial pressures is the same in normal and diseased vascular beds.
Blood flow is determined not only by OPP, but also by vascular tone (resistance). Regulation of blood flow may occur through changes in vascular resistance (vasoconstriction or vasodilation) independently of changes in the OPP. Although the physics of ocular blood flow are the same in nonprimate animal models and in humans, the regulation of ocular blood flow is likely to vary among species because of anatomic differences. For example, in contrast to humans, the rabbit retinal circulation is negligible and the retina is nourished mostly from the choroid. Other differences between animal models and humans, including postural changes from a 4-legged position to upright and differences in circadian activity, may also make it difficult to extrapolate findings concerning the regulation of blood flow from one species to another.
Autoregulation of ocular blood flow is designed to match the metabolic needs of ocular tissues to maintain consistent blood flow despite changes in the OPP. In the retinal circulation, blood flow autoregulation is desirable because retinal metabolic activity and the consequent need for oxygen, nutrients, and waste removal are blood flow dependent. Because the choroidal vascular bed is a high flow bed, with the smallest arteriovenous oxygen difference of any in the body, the extent to which metabolic autoregulation of choroidal blood flow occurs is not clear. However, measurements of the retinal PO 2 gradient in primates show that normal choroid blood flow provides adequate oxygen delivery to the photoreceptor inner segments under light-adapted conditions and marginal oxygen delivery under dark-adapted conditions. Thus, despite the low arteriovenous oxygen difference, the choroid is not over-perfused for retinal oxygen delivery, and even mild reductions in choroidal blood flow can render the photoreceptor inner segments hypoxic. Efficient autoregulation of choroidal blood flow over defined ranges of ocular arterial pressure and IOP has been observed in rabbits. In contrast, in cats and primates in which the radioactive microsphere technique was used to measure ocular blood flow, induction of ocular hypertension resulted in decreased blood flow through the choroid while retinal blood flow remained at normal levels, suggesting a marked difference in autoregulatory capacity between the choroidal and retinal vascular beds. A more recent primate study with laser speckle flowgraphy to measure blood flow demonstrated autoregulation of optic nerve head blood flow at some levels of blood pressure. Studies with laser Doppler flowmetry in humans also indicate optic nerve head autoregulation when IOP is increased stepwise with a suction cup. Moreover, the optic nerve head hyperemic response to flicker light stimulation in animals and humans indicates efficient autoregulatory neurovascular coupling to match metabolism and perfusion.
Autoregulation of both the retinal and choroidal blood flow may make it difficult to modify ocular circulation for therapeutic benefit. Moreover, there may be unforeseen adverse consequences. For example, in a rabbit model, administration of vasodilators to increase blood flow causes an increase in ocular blood volume, which leads to a large, transient spike in IOP that could damage an already diseased optic nerve.
Ischemia and Glaucoma
Ocular nerve head cupping is a hallmark of glaucoma. Although multiple factors are likely to be involved in the etiology of glaucoma, IOP is the best recognized. Laminar cupping may result from the mechanical stress of IOP that causes deformation of the optic nerve head, while prelaminar cupping may result from retinal ganglion cell loss and changes in glial architecture. Other possible etiologic factors in glaucoma include vascular factors, genetic factors, autoimmunity, loss of normal stress responses, and inflammation; furthermore, all of these factors may interact. Glaucomatous optic nerve head damage does not resemble ischemic damage, which is usually characterized by initial pallor of the optic nerve head rather than cupping. Elevated IOP often associated with glaucoma could cause microangiopathy and decreased ocular blood flow. Blood velocity measurements have typically shown a difference between primary open-angle glaucoma patients and normal controls. It is difficult to know, however, whether the decreased blood flow is actually involved in the etiology of glaucoma, or whether it is secondary from a loss of retinal ganglion cells and a decrease in the corresponding metabolic demand for oxygen and nutrients. In other words, is it the cause or the effect?
Findings of elevated concentrations of the vasoconstrictor endothelin-1 (ET-1) in the aqueous humor of glaucoma patients have supported a possible role for optic nerve ischemia in the pathophysiology of glaucoma. The role of ischemia in glaucoma has been investigated in rat, rabbit, and primate models in which ET-1 is administered to chronically decrease optic nerve blood flow. In the rat model, ET-1 administration caused retinal ganglion cell axonal loss, but in vivo imaging showed no optic nerve head cupping characteristic of glaucoma. Optic nerve head cupping does occur in the rat model when IOP is elevated, despite the absence of a well-developed lamina cribrosa. The related question of whether ischemia might increase susceptibility to pressure-mediated damage is difficult to address experimentally and remains to be answered. Inter-species differences also add to difficulties with the interpretation of experimental findings.
The loss of retinal ganglion cells in ET-1 animal models has fueled the hypothesis that ischemia may be involved in glaucoma. However, the damage to retinal ganglion cells in the ET-1 rat model does not appear to be mediated by the vascular effects of ET-1, as no change in blood flow was measured at concentrations of ET-1 that caused death of retinal ganglion cells. Evidence suggests that the death of retinal ganglion cells is mediated by effects of ET-1 independent of, or in addition to, ischemia, including the possibilities of astrocyte proliferation and effects from changes in endothelin B receptor expression.
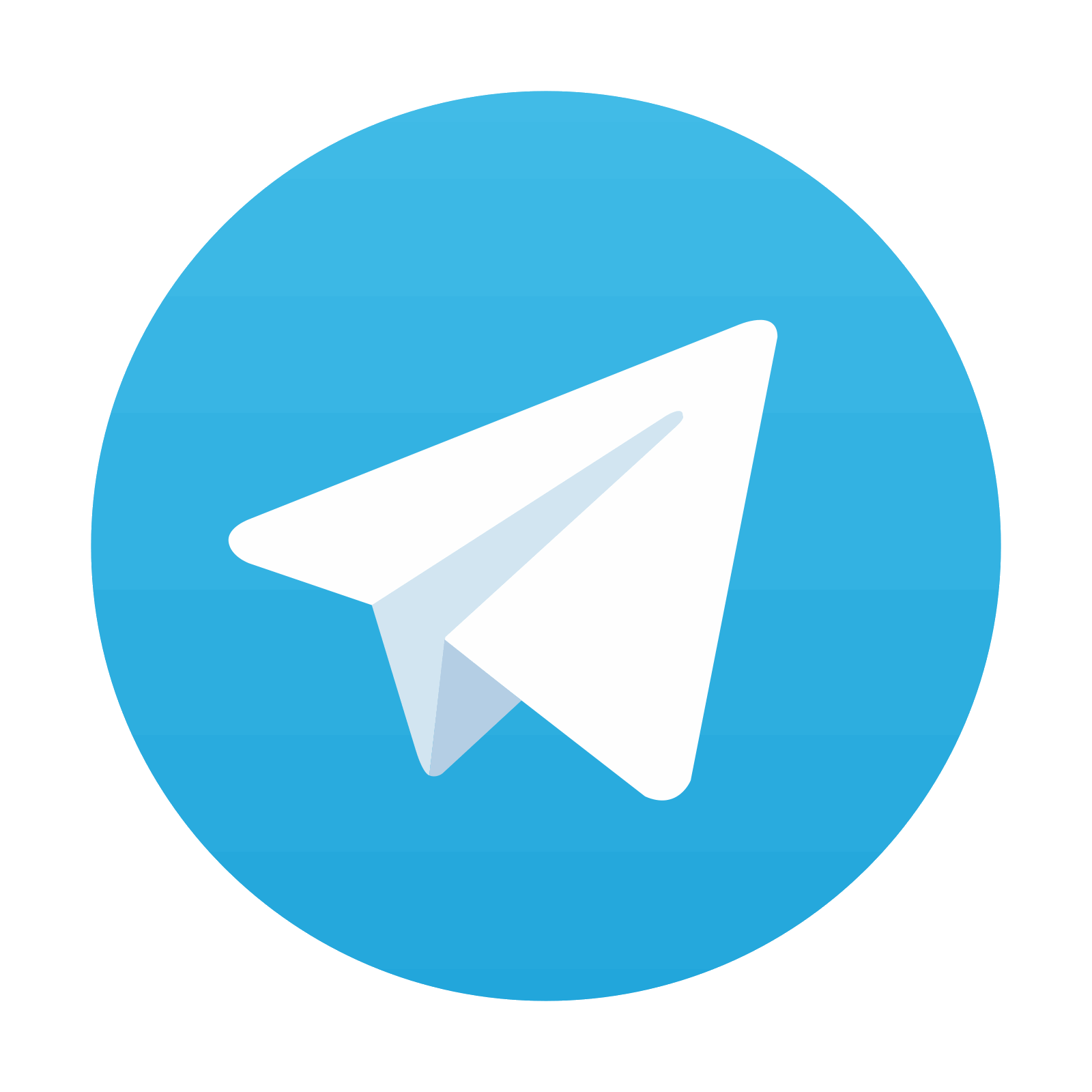
Stay updated, free articles. Join our Telegram channel
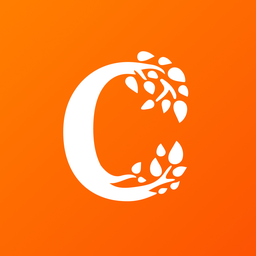
Full access? Get Clinical Tree
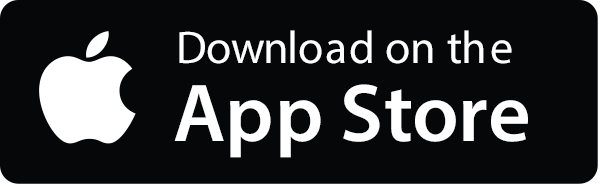
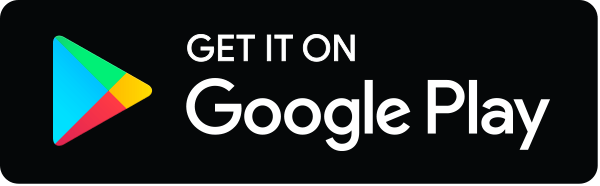