Various types of ionizing radiation may be used therapeutically for benign skull base tumors. Treatment may involve single-dose radiosurgery, or may be fractionated into multiple doses. Designing and implementing a radiotherapy plan that maximizes the therapeutic ratio requires knowledge of the biophysical and radiobiological principles involved in these treatments. These basic radiobiological tenets are discussed in this chapter, with the focus on radiotherapy of benign skull base tumors. Animal and clinical data, however, acquired from the radiation of malignant tumors are necessarily included, as they comprise much of our knowledge of fractionation schedules, central nervous system (CNS) toxicity, and CNS volume effects.
Various benign tumors arise in the base of the skull including epithelial, fibro-osseous, mesenchymal, neurogenic, and vascular varieties. Despite the slow growth of benign skull base tumors, significant clinical symptoms, and even death, may result from compression and dysfunction of adjacent vital structures. The mainstay of treatment of benign skull base tumors is surgical resection. Radiation may be indicated in nonoperable cases or in the postoperative or recurrent setting. The goal of radiotherapy is to provide local control without a high risk of treatment-related toxicity.
Radiotherapy of tumors of the skull base may involve one of several types of ionizing radiation, given as single fraction (most commonly referred to as stereotactic radiosurgery or SRS) or fractionated radiotherapy. An understanding of the biophysical and radiobiological principles involved in these treatments is essential to the design and delivery of safe and efficacious treatment. The purpose of this chapter is to discuss the basic radiobiological principles applied to radiotherapy of skull base tumors.
Types of ionizing radiation
Gamma Rays and X-Rays
Gamma rays and X-rays are electromagnetic radiation with energies in the range of 100 to 2 billion electron volts (eV). X-rays are produced when electrons transition from a higher to lower energy level, usually in the outer shell of heavy atoms and are thus produced outside the nucleus. X-rays may be the products of radioactivity (electron capture) or may be man made from X-ray tubes or linear accelerators, which accelerate electrons onto a heavy metal target producing a continuous spectrum of photon energies up to that of the accelerated electrons. Gamma rays are photons emitted by radioactive nuclei (eg, Cobalt-60) and have a much narrower range of energies than X-rays, 10 keV to 10 MeV. Gamma rays and X-rays are otherwise identical and once produced are indistinguishable.
Protons
The applicability of protons to radiotherapy is based on the physical properties of these particles and the related characteristics of dose deposition in irradiated tissues. Quantitatively, the entrance dose for particle beams is low compared to photons. An unaltered beam will deposit more than 50% of its energy over a 2- to 3-cm narrow path at a depth in water that depends on the beam energy. This peak in energy deposition at depth is referred to as the “Bragg Peak.” The beam may be altered to spread the Bragg peak to conform to the thickness and depth of the volume to be treated. However, the entrance dose is significantly increased in this case ( Fig. 1 ).
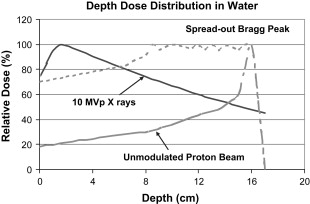
X-rays, gamma rays, and protons are considered to be low linear energy transfer (LET) radiation with roughly equivalent biological effectiveness. Protons have only a slightly higher radiobiological effectiveness (RBE) than Cobalt-60. In practice this small difference is taken into account when prescribing treatment by calculating dose for protons in cobalt Gray equivalents (CGE), whether for single or multiple fractions.
Basic principles of radiobiology
Direct Versus Indirect Effects of Radiation
When tissues are irradiated with low-LET radiation, the vast majority of photons interact with intracellular water molecules by stripping an electron from a hydrogen atom. This results in a fast electron and an ionized water molecule through Compton scattering. The fast electrons interact with surrounding water molecules through further ionizing events. The positively charged water molecules are extremely unstable and dissociate into an H + ion and an OH . free hydroxyl radical. The hydroxyl radical is highly chemically reactive with sufficient energy to break chemical bonds in nearby (within 2 nm) molecules. This indirect effect of radiation acting through the free radical intermediary is responsible for 70% of the damage in irradiated tissues. The remaining damage is caused by the direct effect resulting from fast electrons interacting with the biologically important molecules.
Mammalian Cell Survival Curves
Survival of cells following single doses of ionizing radiation is a probability function of absorbed dose measured in the unit Gray (1 Gy = 1 J/kg absorbed dose). Mammalian cell survival curves obtained following single-dose irradiation in culture ( Fig. 2 ) have a characteristic shape including a low-dose shoulder region followed by a steeply sloped, or more continuously bending, high-dose region. The shoulder region is a reflection of the accumulation of sublethal cellular damage at low doses with lethality resulting from the interaction of two or more such sublethal events. One model considers that DNA is the target molecule for cell killing by ionizing radiation and that a double-strand break in the DNA is necessary and sufficient to cause cell death (defined as loss of ability to divide). Double-strand breaks may result from the passage of a single particle track or by two separate particle tracks causing two single-strand breaks occurring closely in space and time ( Fig. 3 ). Cellular mechanisms of single-strand break repair are efficient, and therefore these represent sublethal damage. By definition, in this model, double-strand breaks are irreparable and lethal. Such a model is described by the linear-quadratic formula
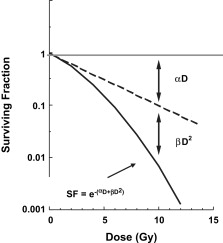
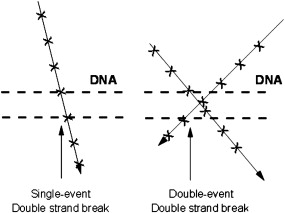
where SF is the fraction of cells surviving a dose, D , of radiation expressed in Gy. α is the coefficient related to single-event cell killing, and β the coefficient related to cell killing through the interaction of sublethal events. α/β is the ratio of the relative contributions of these two components to overall cell kill. The β component, related to the amount of reparable damage, is the more variable of the two coefficients among tissue types. Cell types and tissues may vary in the α/β, resulting in slightly differently shaped response curves ( Fig. 4 A ). The ratio α/β is the single dose at which overall cell killing is equally attributed to these two components (see Fig. 2 ).
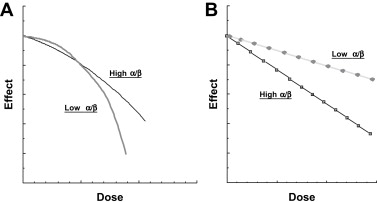
or
Most mammalian cell survival curves are well fit to the linear-quadratic model. Cell survival following a single dose of radiation in vitro reflects the intrinsic radiosensitivity of a particular cell type to a particular type of radiation. There are few in vitro model systems for the study of the radiobiology of benign skull base tumors.
Radiobiology of Fractionated Radiotherapy
A spectrum of fractionation schedules are used to treat benign skull base tumors, ranging from single-fraction radiosurgery to fully fractionated courses of radiotherapy. For fractionated radiotherapy, each dose (fraction) produces similar biological effects (eg, cell survival) given sufficient interfraction interval ( Fig. 4 B). The linear quadratic formula for fractionated doses thus becomes
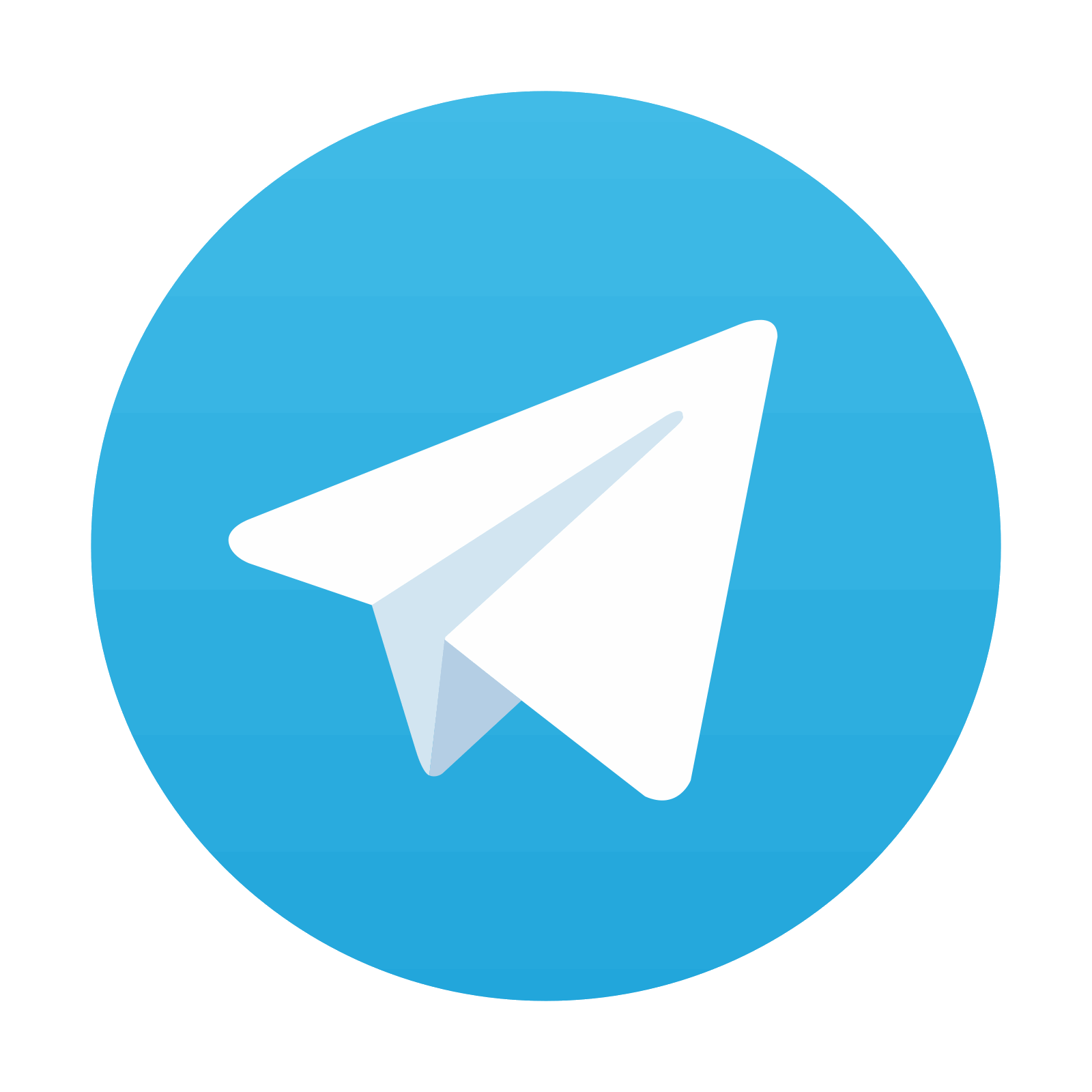
Stay updated, free articles. Join our Telegram channel
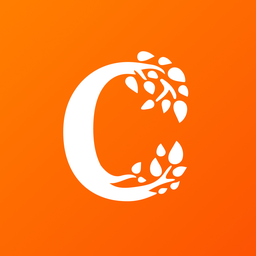
Full access? Get Clinical Tree
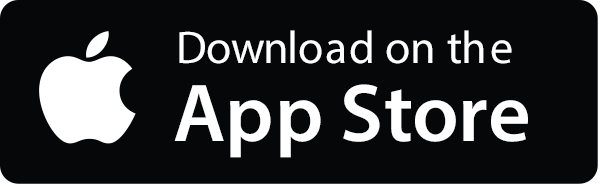
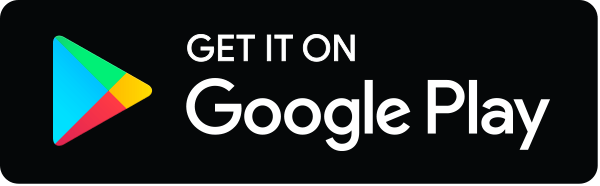