What is DNA?
Deoxyribonucleic acid (DNA) is the basic blueprint for the creation and functioning of an organism. DNA is made up of the nucleotides adenine (A), guanine (G), cytosine (C), and thymidine (T). These nucleotides are lined up in such a way that reading three nucleotides at a time creates a genetic code. Each triplet, also known as a codon, encodes a specific amino acid for the resultant protein. Some triplets are known as stop codons, in that they instruct the body to stop reading the DNA at that point and thus end the process of making the protein. For example, “GAGAAACGGCACTAG” would be read in triplets, where GAG codes for glutamine, AAA for lysine, CGG for arginine, CAC for histidine, and TAG is a stop codon, thus stopping protein formation. DNA exists to make proteins. Proteins may be structural (e.g., collagen, fibrillin), enzymes for metabolic processes, or proteins that regulate other genes. Just as you are reading the words on this page from left to right, a molecule of DNA is said to be oriented from its 5 prime (5’) to 3’ end and is read in that direction.
Some DNA sequences do not encode for proteins. In the past, these areas of the genome were referred to as “junk DNA,” but today it is well established that many of these sequences have important functions, including sequences for ribonucleic acid (RNA) molecules that play a role in transcription, translation, and post-translational protein modification.
1.2 What is a gene?
A gene is a region of DNA that is structured to serve the special function of making RNA and then, usually, a protein. Humans each have approximately 20,000 to 25,000 genes distributed throughout 23 pairs of chromosomes. Chromosomes are identified by number: from largest (1) to smallest (22) with two additional sex chromosomes, X and Y. The first 22 chromosomes are known as autosomes. We have two copies of each autosome. Additionally, females have two X chromosomes, and males have one X and one Y chromosome. Each copy of a pair is called an allele. Each chromosome contains thousands of genes. Each chromosome pair has the same genes although the sequence of each copy of the gene may be different. Each cell in our body, no matter what tissue we are speaking of, has the same chromosomes and the same genes. The difference is that different genes are expressed, or activated, in different tissues. For example, a phototransduction protein is turned off in the lateral rectus but turned on in cells of the retina. Some genes are used in multiple tissues (e.g., the gene that encodes the protein fibrillin), whereas others are more specific to a single tissue.
1.3 What is RNA: transcription and translation?
DNA is transcribed into messenger RNA (mRNA), a single chain made up of the same nucleotides, with the exception of thymidine, which is replaced by uracil (U). The enzyme RNA polymerase catalyzes synthesis of RNA from DNA. Enzymes may modify RNA after it is transcribed from DNA. Each mRNA sequence is created by reading the DNA 3’ to 5’ strand of the double helix and assembling corresponding complement RNA nucleotides. Given that the 3’ to 5’ strand is transcribed, the mRNA strand should directly correspond to the sequence of the 5’ to 3’ DNA strand.
mRNA is converted to protein by the process of translation. Like DNA, the nucleotides of mRNA are read in triplets, each of which codes for one amino acid. As there are 22 possible amino acid choices and a greater number of triplet possibilities (considering the permutations of the four nucleotides), there is redundancy such that more than one triplet can code for the same amino acid. mRNA is decoded by ribosomes, which are made up of ribosomal RNA (rRNA), to produce a specific amino acid chain that will fold into an active protein. Transfer RNA (tRNA) delivers amino acids to the ribosome where they are linked together to form the protein as the mRNA is being read.
Some RNA molecules are not translated into protein. These are called noncoding RNAs. Examples include tRNA and rRNA. Micro-RNAs (miRNAs) and short interfering RNA (siRNA) are other noncoding RNAs that are involved in regulation of gene expression.
1.4 Introns and Exons
Genes have a characteristic structure. Exons are the parts of the gene transcribed into the mRNA product of that gene, and then subsequently translated into protein. A gene can have just a few exons or dozens. Introns are noncoding nucleotide sequences that occur between exons of a single gene. They are transcribed but not translated. Although they do not code for the final gene product, introns are involved with ensuring the proper processing of mRNA, which results in the joining together of transcribed exons on either side of the intron. This process is called splicing. The exon–intron junctions are known as the donor site (5’ end) and acceptor site (3’ end), representing the end of one exon and the beginning of the next exon, respectively. Alternative splicing can generate multiple different functional proteins from a single gene, referred to as isoforms.
A promoter is an area of DNA, usually upstream (5’) from a gene, which is transcribed to produce RNA, and potentially a protein, that initiates and/or regulates RNA transcription of that gene. Promoters are usually 100 to 1,000 nucleotides long.
1.5 Protein functions: building blocks versus enzymes versus transcription factors
Genes that code for proteins have different functions. Some proteins have structural functions, such as myosin, fibrillin, and the many collagens. Other proteins are enzymes that catalyze biochemical reactions such as rhodopsin in phototransduction or rhodopsin kinase. Other genes, sometimes referred to as developmental genes (their expression is necessary in embryonic development), encode proteins that act as transcription factors, which regulate the expression of other genes. Structural genes and genes that encode enzymes are like the instruments in an orchestra. They require a conductor to function in harmony, being expressed at the right time in the right places. Genes that encode transcription factors serve as the conductor by turning on and off other genes in the appropriate places at the correct times.
Every gene is present in every cell of the body, but only certain genes are being used (expressed) in certain cells, thus allowing for unique cell identity. Genes that are used to make a photoreceptor may not be used in the liver and vice versa. Some genes are used in more than one tissue. For example, genes that are involved with the maintenance and function of cilia are expressed in the retina and may also be used in adipose cells, brain cells, and tissues involving hair cells in the ears. When mutated, the resulting disorder may have features involving all of these tissues as seen in Bardet–Biedl syndrome: retinitis pigmentosa, obesity, developmental delay, hearing loss, and other findings. The expression in multiple tissues may be necessary because the structural protein is used in each tissue (e.g., fibrillin in the lens zonules and the aorta) or because a transcription factor regulates different genes in different cells.
1.6 Types of mutations
A change in the nucleotide sequence that leads to a structural or functional disruption of a gene product is called a mutation if there is an associated clinical abnormality. A mutation may involve only one nucleotide (point mutations) or more than one. A missense mutation occurs when the change in a nucleotide results in a different amino acid in the protein primary structure. A nonsense mutation occurs when a nucleotide change causes a codon to change from one that codes for an amino acid to one that indicates protein production to stop, known as a stop codon, thus truncating the protein. There can also be nucleotide insertions or deletions, which cause the reading frame to be shifted (frameshift mutation), resulting in an entire change in amino acids for a whole segment of the protein thereafter until it eventually comes to a stop. Having such a jumbled protein is usually deleterious. Mutations can also be an alteration in chromosomal structure, thus affecting the ability of one or more nearby genes to function normally. One example is the increase in the number of repetitive sequences known as triplet repeats (e.g., CAGCAGCAG) that may normally be present in a gene. This repeat expansion can cause the gene to malfunction, depending on the size of the expansion.
A somatic mutation is one that occurs in a somatic cell, any cell after the fertilized egg (zygote) begins to divide into progeny cells. All descendants of the cell carrying the new mutation will also harbor that mutation. The earlier in development the mutation occurs, the larger the number of cells that are affected. When a person has a mutation confined to a population of cells that derived from a cell that experienced a somatic mutation, the individual has somatic mosaicism: one population of cells with the mutation and the remainder without. A germline mutation originates in a gamete (sperm or egg) so that it is present in the zygote and therefore in all cells in the body. Germline mosaicism may also occur, in which case only some of the egg or sperm population of the transmitting parent has the mutation. Germline mutation is required to transmit a mutated gene to offspring. Somatic mutations are usually not heritable. However, it is possible an individual exhibiting somatic mosaicism in multiple tissues may have mosaicism for their mutation in their gonadal tissue as well and would therefore have a risk of transmitting their mutation to their offspring.
1.7 Mutation versus polymorphism
Despite all of the variations between us, it has been estimated that the difference between the DNA sequences of individuals is only 0.1%. This is equivalent to 30 million nucleotides. These differences in our gene sequences do not cause disease but result in normal human variation such as iris, skin and hair color, height, and variable facial features. Such non–disease-causing variations in our gene sequences are called polymorphisms. By definition, genetic polymorphism is a variant that occurs with a minimum frequency of 1% in a given population.
1.8 Genetic Heterogeneity, Phenotypic heterogeneity, Expressivity, and Penetrance
Genetic heterogeneity means that a single phenotype (e.g., retinitis pigmentosa) may be caused by a number of different genes. Conversely, phenotypic heterogeneity means that different mutations in the same gene may be associated with multiple diseases. For example, mutations in ABCA4 can result in phenotypes such as Stargardt disease, retinitis pigmentosa, cone–rod dystrophy.
Variable expression refers to a difference in severity of the same disorder due to the same gene mutation between individuals. For example, in the same family we might see a patient severely affected with neurofibromatosis, while another is more mildly affected. This is presumably due to polymorphisms in other genes that cause the gene mutation to “act differently” in different people. Expressivity should not be confused with penetrance, which refers to the presence or absence of a particular clinical phenotype associated with a particular gene mutation. A person who has the gene mutation but shows no phenotype is termed “nonpenetrant.”
1.9 Nomenclature
DNA variations are reported with specific notation. A deeper insight can be found at the Human Gene Mutation Database (HGMD) website (http://www.hgmd.cf.ac.uk/docs/mut_nom.html; last accessed July 2016). Changes in genes, which code for proteins, are designated with a “c” followed by the position number of the affected nucleotide in the gene and then the change at that position: c.192C>A, indicates that a cytosine is replaced by an adenine at the 192nd nucleotide position within this coding gene. If a nucleotide change occurs in exon–intron boundaries, a “+” (splice donor) or “–” (splice acceptor) notation can be seen. For example, 123+1C>T means substitution of thymidine for cytosine one nucleotide beyond the splice donor site, which starts at nucleotide 123 from the 3’ end of the coding sequence of the gene. Likewise, 247–2A>G means substitution of guanine for adenine two nucleotides upstream from the splice acceptor, which ends at nucleotide number 247. In other words, the next exon begins at nucleotide position 247.
Protein variations are annotated with a “p” that stands for “protein” followed by the amino acid change and position of the change in the protein: p.Arg650Cys indicates that the amino acid arginine at position 650 in the protein is replaced by the amino acid cysteine. This is a missense mutation. The nomenclature proposed in the HGMD reports the same change using the single letter, which is designated for the amino acid: R650C indicating the change from arginine (R) to cysteine (C).
Nonsense mutations are annotated by the nucleotide change in the coding DNA, but the resultant truncation of the protein is indicated by using “X,” “*,” or “ter” for the result of the change. For example, c.374 C>G indicates that a cytosine is changed to a guanine at nucleotide 374 of the gene. If this was the last nucleotide in the codon TAC, which encodes for tyrosine, it would now be TAG, which is a stop codon. The resulting change in the protein would be denoted as Y109X, p.Tyr109*, or p.Tyr109ter, indicating that the tyrosine is no longer added to the protein at this position. Rather, the protein is truncated at this point in the amino acid chain (▶ Fig. 1.1). When there is an insertion of one or more nucleotides to DNA, the inserted nucleotides are indicated in the nomenclature. This can produce a change that may lead to a mutation. An insertion can also occur at the chromosome level, but instead of nucleotides, large pieces of chromosomes are inserted. On the other hand, a deletion is when a sequence of DNA or a piece of chromosome is missing, leading to an imbalance that may express as a mutation or absence of protein expressed by the genes that were deleted.
Fig. 1.1 Codon wheel, showing the combination of nucleotides to form different amino acids.
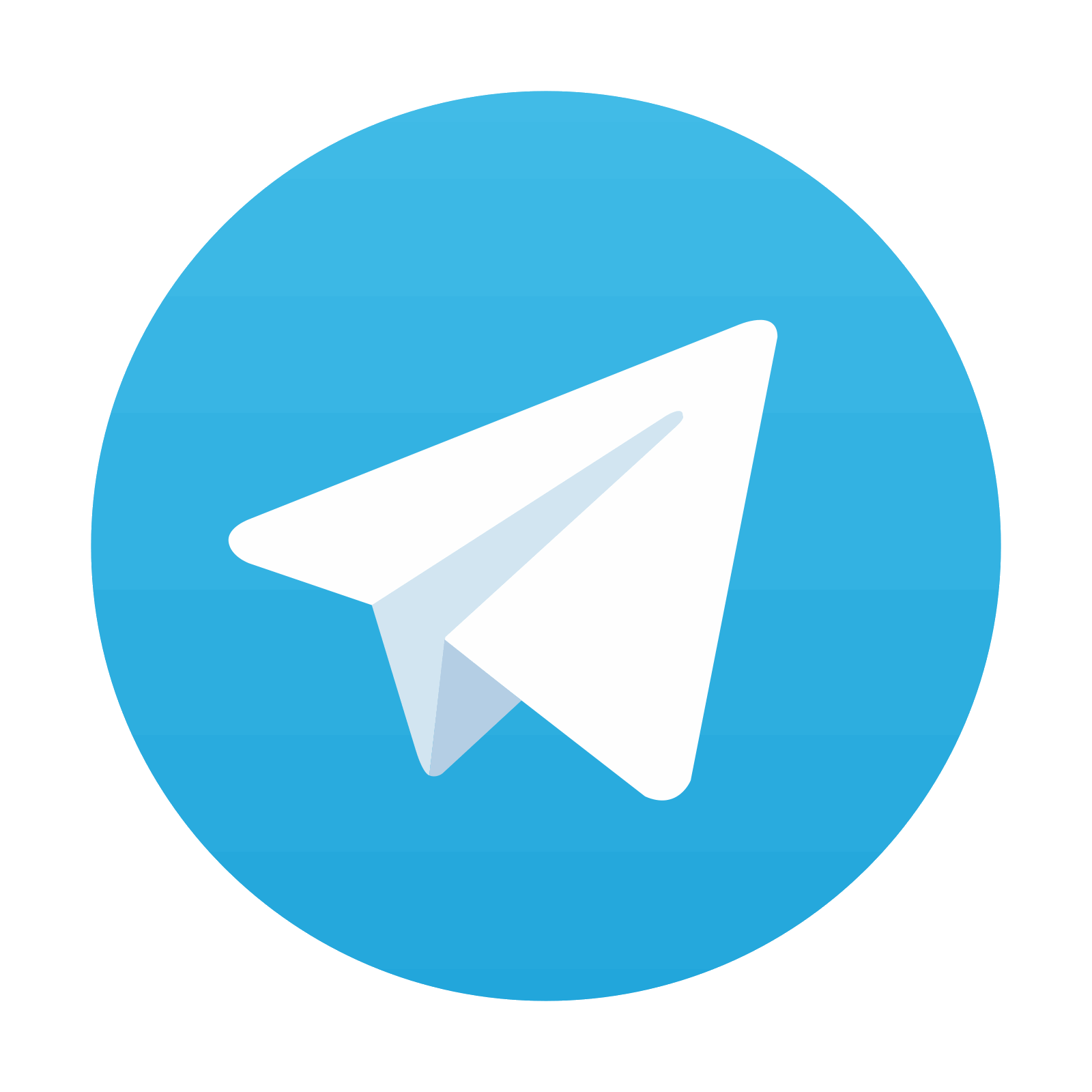
Stay updated, free articles. Join our Telegram channel
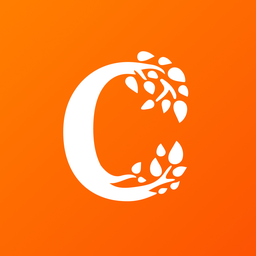
Full access? Get Clinical Tree
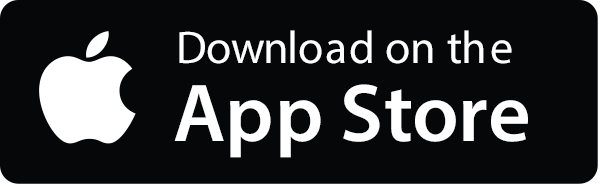
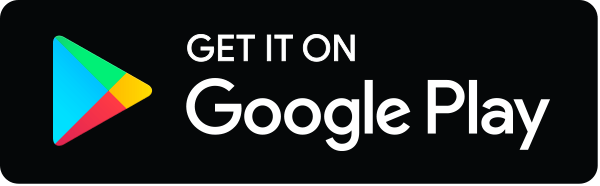