Physical acoustics encompasses a huge range of topics, many of which are not covered in this text. The information presented here is selected to serve as a foundation for the study of speech acoustics (Chapters 8–11), auditory anatomy and physiology (Chapter 13), and auditory psychophysics (Chapter 14). Readers interested in a full, technical treatment of acoustics should consult the classic textbook by Beranek (1986, originally published in 1954). Entertaining histories of acoustics as a scientific discipline have been published by Hunt (1978) and Beyer (1999).
Acoustics has been studied for centuries (Hunt, 1978). Over these many years a rich foundation of knowledge has evolved that is not specific to individual, contemporary studies in the scientific literature. This chapter does not cite a large or even modest number of publications, but rather derives its presentation from a composite of textbook material written by Beranek (1986), Blackstock (2000), and Kinsler, Frey, Coppens, and Sanders (2000).
This chapter addresses a series of questions. Answers to these questions provide a foundational understanding of speech acoustics and audition. The questions are as follows:
1. What are pressure (sound) waves, and how can they be measured?
2. What is sinusoidal motion, and which measurements are relevant to its description?
3. What are complex acoustic events, and how are they related to sinusoids?
4. What is the phenomenon of resonance, and how is resonant frequency determined in mechanical and acoustical systems?
5. What does it mean to say that a resonator “shapes” an input?
6. What is the difference between a resonator and a filter?
The leading acoustics organization in the world is the Acoustical Society of America (ASA), founded in 1929. Roughly 35 scientists attended the initial 1929 meeting of the Society (that’s the number counted in a photograph taken of the attendees at the meeting). Today, ASA has over 7,000 members, who study all aspects of acoustics. The Society encourages open exchange of ideas and education in acoustics. It wasn’t always so. The science of acoustics goes way back to the Ionian philosopher Pythagoras (ca. 575–495 BC), the same fellow after whom a famous geometry theorem is named. Pythagoras enjoyed a sort of guru status, and his followers decided that their investigations should be kept secret to protect their intellectual sanctity. We know the Pythagoreans made numerous observations about acoustical events, but alas, we only learned about these through indirect sources—leaks in the system. So, the first Acoustical Society was really a Secret Acoustical Society.
This section considers the nature of pressure waves. It discusses the forces that govern such waves, how local changes in air pressure occur, how pressure waves propagate through space, and how such waves can be measured and represented.
The Motions of Vibrating Air Molecules Are Governed by Simple Forces
Sound can be defined as the propagation of a pressure wave—a sound wave—in space and time. Clarification of this definition requires a precise definition of exactly what is meant by the term pressure wave.
Sound is always propagated through some medium. The various media that conduct sound waves (e.g., water, steel, air) are composed of molecules that are compressible, the degree of compressibility depending on the medium. In other words, molecules of these media may, under the influence of a force, be displaced away from their rest positions (where “rest positions” refers to the positions when no forces are applied to the medium) and moved closer to nearby molecules. The resulting “bunching up” of molecules, relative to their distribution at rest, shows that the medium is compressible.
The molecules of all sound-conducting media share the characteristics of elasticity and mass. Elastic objects oppose displacement, and do so with greater magnitude as they are moved farther from their rest positions. A common example of this is a rubber band. When the rubber band is completely collapsed, it is in its rest position. As the rubber band is stretched, it becomes stiffer. Increasing the stretch on a rubber band not only increases its stiffness, but makes it more difficult to stretch. Massive objects oppose being accelerated and decelerated (that is, they have inertia: “a body in motion tends to stay in motion”), and do so with greater magnitude as they become more massive. The effect of mass on motion is easily appreciated by the speeding automobile that does not stop instantly when the brakes are applied.
Opposition to displacement (elasticity) and acceleration/deceleration (inertia) result in energy storage. This stored energy can be expressed as motion of the object even in the absence of an external force. This topic is taken up in greater detail below.
The sound-conducting medium of interest for speech is, at least in most cases, air. The number of molecules in a cubic inch of air, referred to as the density of air and symbolized with the Greek letter rho (ρ), is approximately 4 × 1023. The density of molecules in water is about four times greater than that in air, and in steel about eleven times greater than air.
With air as the medium, a schematic drawing1 can be used to illustrate the concepts mentioned above. Imagine, as shown in Figure 7–1, four columns of air molecules (labeled 1, 2, 3, 4) extending across some distance. Five rows, labeled A, B, C, D, E, show the state of these molecules at successive moments in time. Row A (hereafter, time A) shows the molecules at rest, with no external forces applied to them. This rest position is indicated across all times by the dashed lines extending downward throughout the four columns of molecules. Note the even spacing between the molecules across time A.
Molecules at rest are still in motion (this small motion is referred to as Brownian motion), but the average spacing between any pair of molecules will be roughly equivalent, as shown in Figure 7–1, time A. Because the molecules at time A are not being displaced from their rest positions and thus not being accelerated, they store no elastic or inertial energy (other than the negligible energies stored due to Brownian motion). At time B, the molecule in column 1 is subjected to a force (indicated by the rightward directed arrow) that moves it rightward, away from its rest position and in the direction of the molecule at position 2. For present purposes, a force can be thought of as any push or pull applied to an object. As the molecule moves farther from its rest position, it opposes increasing displacement by generating an increasing recoil force, which is exerted in the direction of the rest position, or the direction opposite to its current motion. Stated in a different way, the original force of the rightward push is transferred to the air molecule, whose rightward displacement is increasingly opposed by the tendency of the molecule to recoil back to its rest position. The current motion continues away from the rest position because the original force of the push still exceeds the recoil force. The recoil force is the stored energy resulting from the displacement of the molecule from its rest position. Recoil is a hallmark of all elastic objects.
At position 2, time B, several things happen. First, the spacing between molecules 1 and 2 is now minimal compared with their spacing at rest (time A). At position 2, time B, the molecules are bunched up, and molecule 1 bumps molecule 2, just as the push originally bumped molecule 1 to get this process under way. Second, the molecule that was originally pushed to start the process and is now displaced relatively far from its rest position generates a strong recoil force to return to its rest position. At some point, the recoil force equals and then exceeds the original force and thus stops its rightward motion and begins to move leftward, back toward the rest position. Assume that the bump of molecule 2 by molecule 1 occurs at the same time as the recoil force of molecule 1 becomes greater than the original force on it. Molecule 1 therefore imparts a displacing force on molecule 2 and generates its own force to return to its rest position.
Figure 7–1. Schematic drawing of the vibration of air molecules around their rest positions. Rest positions of four molecules are shown at time “A” at positions 1, 2, 3, and 4. Time is indicated by the rows A, B, C, D, and E. A pressure-measuring device is located at the rest position of molecule 2. See text for details.
At time C, the molecule from position 1 is headed back toward the rest position (leftward-directed arrow). The molecule from position 2, having been bumped by the position 1 molecule, now moves away from its rest position and in the direction of the molecule at position 3 (rightward-directed arrow). At time D, the molecule from position 2 is bumping into the molecule at position 3, and is also about to reverse its direction—just like the situation discussed above for time B, position 2. The molecule from position 1, however, has gone through its rest position, and is displaced far to the left—note at time D the wide separation of the molecules from positions 1 and 2. Clearly, the elastic recoil forces caused the molecule from position 1 to move back toward the rest position at time E, but why doesn’t the motion stop at the rest position—why has it passed the rest position at point 1? The answer is that inertial forces do not allow the molecule to “stop on a dime” at the rest position, but drive the molecule through rest and to the rightward extreme. The molecule, which has mass and opposes acceleration and deceleration, does not decelerate instantly to stop at the rest position, but rather continues its motion through that point in space. As the molecule passes through the rest position and continues to move away from it, it is once again stretched and develops recoil force. The molecule continues this motion until the recoil forces again overcome the forces driving the molecule away from its rest position (primarily inertial). Once again, the motion is reversed, and the molecule heads back in the direction of the rest position. And once again, the motion does not stop at the rest position because of inertial forces; the molecule continues to move back and forth around the rest position.
It is important to understand that the forces maintaining the back and forth motion of the molecule around the rest position are a product of the motion itself: recoil and inertial forces result from the motion of the molecule. These forces are intrinsic to the motion of the molecule, rather than imposed from an outside source (as was the case with the bumping force that initiated the motion).
After the original application of the force at position 1, therefore, the back and forth motion is maintained by energy stored by the molecule itself. If the molecules were vibrating in a frictionless medium, in which no energy was lost because of heat generation, the back and forth motion would continue indefinitely. Realistically, heat loss due to air molecules rubbing against each other and other surfaces eventually cause the back and forth motion to die out if external forces are not continuously reapplied to the air molecules. However, the principles of recoil and inertia forces still apply to a dying-out motion. The frictional forces compete with the recoil and inertial forces and, in the absence of external forces (such as another bump), eventually dominate the latter two and end the motion.
The Motions of Vibrating Air Molecules Change the Local Densities of Air
The motion of air molecules and the forces that control that motion have been described. How can this description lead to an understanding of pressure waves? Assume that a device for measuring air pressure is placed at position 2 in Figure 7–1. Pressure can be defined as the force exerted over a unit area (P = F/A) and is proportional to the density of air. When air molecules are more densely packed in some unit volume, they collide with each other more frequently and generate more force and more pressure within that volume. Conversely, when air molecules are less densely packed, the collisions are less frequent and the pressures are relatively lower. At time A in Figure 7–1, there are no external forces applied to the air molecules and the density of air is that associated with air at rest (recall the 4 × 1023 figure given above). The pressure measured at time A, position 2 (or at any other position at time A, because the density of air at rest is the same at any spatial location) is referred to as atmospheric pressure. The actual value of atmospheric pressure is not important to the current discussion,2 but the reference function of this pressure is important. In the following discussion, atmospheric pressure (symbolized hereafter as Patm) is considered as zero (0) pressure. At time B, the schematic drawing shows two molecules close together at position 2, indicating a relatively denser packing of air molecules at that position compared with time A. The pressure measuring device at time B, position 2 measures a higher pressure than at time A because the denser packing of molecules involves more frequent collisions and higher forces. Any time a pressure is above the reference pressure Patm, it is referred to as positive pressure. The more tightly packed the molecules, the more positive the pressure.
At time D, position 2, the first and second molecules are widely separated in space. This represents a case where the density of air is less than it is at Patm. The pressure-measuring device records a value below Patm, which is a negative pressure. The less tightly packed the molecules, the more negative the pressure.
These positive and negative pressures are not positive or negative in any absolute sense. They are only positive (above) or negative (below) with respect to the reference pressure Patm. Figure 7–2 shows the result of the continuous bunching up and spreading apart of the air molecules schematized in Figure 7–1.
The three rows labeled A, B, D in Figure 7–2 correspond to the same rows in Figure 7–1 (rows C and E from Figure 7–1 have been omitted from Figure 7–2), indicating three different times in the evolving history of air molecule movement. At time A, each of the multiple dots represents an air molecule, and the collection of these molecules is shown evenly distributed across space, as expected if the medium is not affected by an external force. The pressure at any point throughout the distribution, measured at any location in space from the left to the right edge of the dot display, is Patm.
At time B, this distribution has been changed to one in which regions of low and high density alternate across space. The heavily shaded areas represent high densities (as when two molecules are immediately adjacent, Figure 7–1, time B, position 2), and the lightly shaded areas represent low densities (Figure 7–1, time D, position 2). At time D, regions of high and low densities again alternate across space, but their locations have been reversed from those at time B. In other words, for a given point in space (such as position 2), what was a high-density and high-pressure area at time B is a low-density, low-pressure area at time D. Areas of high density and high pressure are called areas of compression or condensation3; areas of low density and low pressures are called areas of rarefaction.
Pressure Waves, Not Individual Molecules, Propagate Through Space and Vary as a Function of Both Space and Time
Recall what was said earlier about the motion of individual air molecules. They move around a rest position, the movement resulting from inherent elastic and inertial forces. The molecules themselves, however, do not propagate across space. What moves across space is the pressure wave, shown in Figure 7–2 from left to right as the sequence of high and low densities (pressures), or the sequence of compression (condensation) and rarefaction areas. Because these alternating regions of high and low pressures are the result of the back and forth movement of air molecules, which alternately bunches them up and spreads them apart, a given point in space sometimes has high pressure (such as time B, position 2 in Figure 7–1), and sometimes has low pressure (time D, position 2 in Figure 7–1). Thus, a pressure wave extends across space at a specific instant in time (examine Figure 7–2, across time B or D) and varies in time at a specific point in space (Figure 7–2, compare times B and D at any point in space).
Figure 7–2. Schematic drawing of pressure waves, distributed in space and time. A, B, and D correspond to discrete times depicted in Figure 7–1. At a given point in space, pressure changes over time.
When a pressure wave moves away from the source of the sound waves (the origin of the forces that initiated the displacement of air molecules), the alternating regions of high and low pressures often project more or less in a straight line, as shown from left to right in Figure 7–2. These kinds of sound waves are called plane waves, because the sequence of high and low pressures can be thought of as a series of pressure “slices,” or planes, extending away from the source. The pathway followed by plane waves may vary as a function of frequency, as discussed below. For the present discussion, only plane waves are considered, but other kinds of pressure waves are possible (for example, those moving sideways from a source, rather than in a straight line away from the source: see comment on this in Chapter 11).
The Variation of a Pressure Wave in Time and Space Can Be Measured
There are specific measures of the temporal (time) and spatial (space) variation of pressure waves. A firm grasp of these measures is essential to understanding important aspects of the acoustic theory of vowel production, covered in Chapter 8.
Figure 7–3A redraws a portion of the molecule motion shown in Figure 7–1. Of interest is the motion of the first molecule, shown in Figure 7–3A as a shaded circle. As in Figure 7–1, space (distance) is shown from left to right, and time from top to bottom (times A, B, C, D, E, occurring in succession). The numbers 1, 2, 3, 4, and 5 above the filled circles show the position of the first molecule at five discrete points in time, starting from the molecule at the rest position “1” and ending back at the rest position “5.” At time B (molecule labeled “2”), the molecule is immediately adjacent to the next molecule, which creates a region of compression, or high pressure, at the point in space indicated by the upward-pointing arrow. The pressure at this point in space becomes somewhat negative at time C (molecule labeled “3”), more negative yet at time D (molecule labeled “4”), and returns to Patm at time E (molecule labeled “5”).
The motion of the filled-circle molecule is shown as a function of time in Figure 7–3B. When any magnitude (such as displacement, pressure, speed, and so forth) is shown as a function of time, the plot is called a waveform. This waveform shows the position of the molecule from rest position (1), to the rightward extreme (2), the return through the rest position (3), at the leftward extreme (4), and finally back at the rest position (5). The discrete positions are connected to illustrate the continuous motion of the molecule throughout the vibratory cycle. This waveform shows one complete cycle of motion of the molecule. If the molecule continues to move after point 5, it repeats the 1 through 5 sequence of motions and produces another cycle with the same characteristics.
Figure 7–3. A. Schematic drawing of the vibration of an air molecule (filled circles) around its rest position. Numbers above the molecule indicate five successive points in time throughout its vibratory cycle. B. Plot of the position of the filled-circle molecule and the pressure measured at the location of the second molecule during one vibratory cycle.
The y-axis of the waveform in Figure 7–3B is also labeled pressure because it is easy to show that air pressure (measured at position 2) and position of the first molecule change in the same way over time. The rightmost extreme of molecule movement (“2” in Figure 7–3A) is also the time when air compression is maximum at the pressure measurement point, the leftmost extreme (“4”) will occur at the same time as a rarefaction at the pressure measurement point, and the rest positions (“1” and “5”) will be associated with Patm. Positions between these discrete times will have pressures somewhere between compressions and Patm or rarefactions and Patm. Thus, the waveform in Figure 7–3B shows the temporal variation of the pressure wave. The period (T) is the time taken to complete one full cycle of motion; T is given by the formula: T = 1/f, where f = frequency. The filled-circle molecule is plotted as a continuous function of time as shown in Figure 7–3B. Figure 7–3A only indicates 5 discrete points in time, but Figure 7–3B shows every possible position of the molecule from time A through E, and how those positions change over time. Time is shown on the x-axis and molecule position (or air pressure) is shown on the y-axis. The horizontal line separating the upper and lower halves of the waveform indicates the rest position of the molecule, that is, when pressure = Patm. The numbers 1, 2, 3, 4, and 5 given in Figure 7–3A are indicated on the time plot in their appropriate locations. According to the time scale shown on the waveform in Figure 7–3B, it takes .01 second to complete one cycle of molecule motion. For this waveform T = .01 second (s) or T = 10 milliseconds (ms).
In this example, .01 s and 10 ms are the same value, but expressed in different units. In speech and hearing applications, it is typical to express time units in ms. For reference purposes, 1 s = 1000 ms, 0.1 s = 100 ms, 0.01 s = 10 ms, and 0.001 s = 1 ms. In some hearing applications (see Chapters 13 and 14), time may be expressed in units as small as microseconds (μs; 1 μs = 0.000001 s).
The time variation of a repeating, cyclic motion, like the one shown in Figure 7–3B, can also be expressed in terms of frequency, symbolized with an f. Frequency (f) is the inverse of period (T):
The units for frequency are hertz, abbreviated as Hz, which stands for “cycles per second.” The number resulting from the formula indicates how many complete cycles of vibration occur in one second. When formula (1) is applied to the 10-ms period in Figure 7–3B, the result is f = 1/10 ms or f = 1/.01 = 100 Hz.
Because frequency (f) is the inverse of period (T), as one variable (e.g., f) increases, the other (e.g., T) decreases. Longer periods are associated with lower frequencies, and shorter periods are associated with higher frequencies. Figure 7–4 shows the relationship between frequency and period ranging from 1.0 ms to 10.0 ms. For this graph, period is plotted on the x-axis, and is changed in steps of 0.5 ms (i.e., 1.0 ms, 1.5 ms, 2.0 ms, 2.5 ms . . . 10.0 ms). The corresponding frequency values, computed by f = 1/T, are plotted on the y-axis. The inverse relationship between the two expressions of cyclic variation is clearly seen in the way the plotted points descend on the y-axis as the value on the x-axis increases. Note also that the relationship between T and f is not linear. In a perfectly linear relationship between two variables, equal-sized change in one variable is accompanied by equal-sized change in the other variable. If the relationship between T and f were perfectly linear, each 0.5-ms step in T would be accompanied by a constant change in f. In Figure 7–4, however, the frequency changes (y-axis) accompanying the 0.5-ms steps in the region of 10 ms (x-axis) are much smaller than the frequency changes accompanying 0.5-ms steps in the region of 1.0 ms. This is an example of a curvilinear relationship between two variables. For instance, the 0.5-ms change between 9.0 and 9.5 ms on the x-axis produces a change in f of approximately 6 Hz, but the same 0.5-ms change between 2.5 and 3.0 ms produces a change in f of more than 65 Hz.
Figure 7–4. Plot of frequency versus period that shows the inverse and nonlinear relationship between the two variables.
In Figure 7–2, pressure waves were depicted as alternating regions of high and low pressures extending across space. Portions of two pressure waves are shown in Figure 7–5, one corresponding to a frequency of 100 Hz, the other corresponding to a frequency of 1000 Hz. The x-axis is labeled as distance and is scaled from 0 to 500 centimeters (cm) (equivalent to roughly 0 to 195 inches). The portions of the pressure waves for both frequencies shown in Figure 7–5 consist of sequences of high- and low-pressure regions. The high-pressure regions are shown as the more heavily shaded areas, indicating relatively dense packing of air molecules, and the low-pressure areas are shown as lightly shaded, indicating the relatively sparse packing of molecules. It is immediately apparent that one complete variation across space between high and low pressures associated with the 100-Hz sound covers a greater distance than the corresponding variation across space for the 1000-Hz sound. The measurement of spatial variation of a pressure wave is called the wavelength, which is the distance covered by a high-pressure region and its succeeding low-pressure region (or by a low-pressure region and its succeeding high-pressure region). Wavelength is symbolized by the Greek letter lambda (λ), and is shown for both pressure waves in Figure 7–5 as extending from the leading edge of the first high-pressure region (i.e., the position in space where the pressure first becomes positive relative to Patm) to the leading edge of the next high-pressure region. In accord with the definition given immediately above, wavelength includes the distance covered by the first high-pressure region and its succeeding low-pressure region.
Wavelength has an inverse relationship to frequency. The higher the frequency, the shorter the wavelength. This acoustic law is illustrated in Figure 7–5 where the 1000-Hz sound has a much shorter wavelength than the 100-Hz sound. The formula for wavelength is: where f = frequency and c = the speed of sound in air, which is a constant and has a value of approximately 33,600 cm/s (roughly 1100 ft/ s). If the values of the frequencies shown in Figure 7–5 are plugged into the formula along with the value for the constant c, the wavelengths for 100 Hz and 1000 Hz are approximately 336 cm (100 Hz) and 33.6 cm (1000 Hz), respectively.
Figure 7–5. Illustration of the measurement of wavelength. Wavelength is the distance covered by a cycle of pressure variation. This distance is marked as 1λ on the 100-Hz and 1000-Hz signals. Wavelength is longer for the 100-Hz signal. The bottom right inset shows pressure variation plotted as a function of distance.
Note also that the “boundaries” of the low- and high-pressure regions in Figure 7–5 are marked as Patm. As described above, this indicates that as the pressure varies across space, there are points where the pressure is equal to Patm. The pressure varies across space by going above Patm, below it, above it, and so on. The changes in pressure across space are gradual. The graded nature of these pressure changes is illustrated in Figure 7–5 by the continuous changes in the shadings of the compression and rarefaction regions, with the heaviest shading occurring at the most positive pressure and the lightest shading at the most negative pressure.
The continuous changes in pressure across a single wavelength are also shown in the inset to Figure 7–5, where pressure (y-axis) is shown as a function of distance (x-axis). The horizontal line in this inset is Patm, the reference pressure. Pressure increases relative to Patm (“1” in the inset) until it reaches a positive peak, then decreases and passes through Patm (“2” in the inset) as it goes to the negative peak, then reverses again to return to Patm (“3” in the inset). This is a different way to plot the pressure waves shown in the main part of the figure.
The concept of wavelength is critical to understanding why different configurations of the vocal tract (the “tube” of air shaped by the articulators, extending from vocal folds to the lips) result in different spectra for different vowels (see Chapter 8). Wavelength is also an important concept in understanding the variation of auditory sensitivity as a function of frequency, which is explained in large part by the resonance of the ear canal which is an air-filled tube closed at one end (see Chapter 13).
Wavelength and Direction of Sound
Pressure waves that are primarily plane waves move in a straight line away from their source. When sound waves encounter an object along their path, however, straight-line propagation may change. In general, frequencies with very long wavelengths may bend around objects in their path, whereas frequencies with very short wavelengths do not bend and may strike the object. The short wavelengths cause pressure variations to reflect off the object. These pressure reflections interact with the original pressure wave as well as with the multiple reflections.
The relationship between wavelength and objects within the path of a pressure wave is more precisely related to the respective magnitude of the wavelength and the size of the object in its path. If the wavelength of a pressure wave (or of components of a pressure wave with many frequencies; see below) is substantially greater than the size of the object—say, the diameter of a human head—the wave will bend around the object. The same pressure wave, with the same wavelength, may not bend around a massive building.
As discussed in Chapter 14, the issue of wavelength and objects within the path of a pressure wave is relevant to the acoustic information used by humans to localize a sound source in space. If the typical head diameter of the adult female head is roughly 56 cm, wavelengths associated with frequencies of 500 Hz and below can bend around the head (λ = c/f; 33,600/500 = 67.2 cm).
Pressure Waves: A Summary and Introduction to Sinusoids
The motions of air molecules can produce pressure waves, which are the basis of sound. Pressure waves vary across space and time, and both variations can be described and measured using simple mathematics.
To this point, the examples and explanations of these events have been schematic, or simplified to some degree. For example, very little has been said about the causes, or sources, of air molecule motions and resulting pressure waves. Moreover, the motions discussed so far have been very simple, whereas many acoustic events—such as speech—involve complex vibrations of air molecules caused by the motions of complex sources. Even the most complex vibrations, however, can be broken down into a set or group of the simple vibrations described above. It is also the case that a complex vibration can be generated by taking a set of these simple motions and adding them together; complex acoustic events can be created by combining many individual simple acoustic events. The understanding of simple vibrations is the basis for understanding the more complex vibrations observed in most acoustic events.
The simple motions and resulting pressure waves discussed above are called sinusoidal motions and waves. Sinusoidal motions are the simplest form of vibration. More complex vibrations can be broken down (“decomposed”) into a set of individual sinusoids. The inverse of this decomposition is that complex acoustic events occur when a group of sinusoids are combined together. A formal description of sinusoidal motion is presented next, along with the idea that complex sounds are the sum of their component sinusoids.
Speed of sound in air varies as a function of air temperature, and to a lesser degree as a function of humidity and altitude. The higher the temperature, the faster the propagation of sound waves. Students will find slightly different values used in different texts. Thirty-three thousand six hundred centimeters per second is roughly the value that is measured at 0 degrees Celsius (32 degrees F). Speed of sound in air increases as temperature rises because molecules move faster at higher, compared with lower, temperatures. Speed of sound also varies with the nature of the conducting medium. Sound propagation is about 4 times faster in water compared with air, and about 11 times faster in steel compared with air (everyone knows the trick of putting an ear to a railroad track to “hear” an oncoming train that is far away from your ear). The general rule is: the denser the medium, the faster the sound conduction.
In the foregoing discussion of pressure waves, the motion of air molecules was described as governed by simple laws of elasticity and inertia. The effects of recoil and inertial forces produce the oscillation of the molecule around a rest position. This sinusoidal motion (also called simple harmonic motion) has a simple conceptual and mathematical basis.
Sinusoidal Motion (Simple Harmonic Motion) Is Derived from the Linear Projection of Uniform Circular Speed
Imagine, as shown in Figure 7–6A, a circle of some arbitrary radius with its four right angles defined by the radius lines AB, BC, CD, and DA. The filled dot at point A indicates an object that rotates continuously around the circumference of the circle, in a counterclockwise direction. When the object rotates around the circle, it does so with uniform circular speed (UCS). In UCS, the rotating object crosses equal angles in equal amounts of time. In the example shown in Figure 7–6A, this means that the time it takes the rotating point (filled dot) to travel from point A to B will equal the time it takes to travel from point B to C, point C to D, and point D back to A. This is consistent with the definition of uniform circular motion given above, because the angles defined by lines AB, BC, CD, and DA all equal 90 degrees and each 90-degree angle (or each 45-degree angle, or 30-degree angle, and so forth) is traversed in an equal amount of time.
Sinusoidal motion can now be defined as the linear projection of uniform circular speed. This is illustrated in Figure 7–6B, where one-half of the circle has been partitioned into four angles of 45 degrees each. Alongside the circle is a vertical line, the top and bottom of which have the same location as the top and bottom of the circle (point C is located at the top of the circle). At each point along this half-circle motion, a horizontal dashed line has been drawn to intersect the vertical line. The horizontal lines project the labeled points (points A through E) along the circle to the vertical line—hence the expression “linear projection of UCS.” Remember that each of the 45-degree angles shown in Figure 7–6B is traversed by the rotating object in equal amounts of time.
The result of the linear projection of UCS can be best appreciated by comparing the points on the vertical line to the corresponding points on the circle. The projection of point A on the circle results in point A′ on the line, which divides the line into upper and lower segments of equal length. Point B on the circle projects to point B′ on the line, and point C on the circle projects to point C′ on the line. The interesting comparison here is the length of the linear segment A′-B′ to B′-C′. Clearly the segment A′-B′ is longer than the segment B′-C′, yet these two segments result from the projection of points spanning equivalent arcs. In other words, when the circular motion involves equal distances (that is, motion covering equal angles), the linear projection of the points defining those angles does not yield equal linear distances.
As the point moves from point C to point E, its linear projection is superimposed on the same line segment (A′-C′) originally covered when the point rotated from A to C. The result of this projection is now from C′ to A′, which is the reverse of the original projection. As the rotating point moves from E to F, the linear projection extends from A′ to F′, and the projection of the rotation from F to A is superimposed on the A′-F′ line segment, but now in the opposite direction.
Discrete angles on the circle illustrate the linear projection of UCS, but as the point rotates about the circle, the angles change continuously as they are projected to the line segment. The linear projection of a complete rotation around the circle looks very much like the motion of the air molecule in Figures 7–1 and 7–3. A′ on the linear segment is the rest position, whereas C′ and F′ are the extremes of the linear displacement. One complete cycle involves motion from the rest position A′ to the one extreme (C′), back through the rest position to the other extreme (F′), and then back to the rest position. In fact, if the line segment in Figure 7–6B is turned on its side with C′ to the right and F′ to the left, the motion described by the linear projection of the rotating point is the same as the motion of air molecules described above.
Figure 7–6. Derivation of sinusoidal motion from the linear projection of uniform circular speed. A. Filled dot moving around larger circle at uniform circular speed which means it crosses equal angles in equal time intervals. B. Projection of points along the circumference of the larger circle to a line segment, showing how projection from equal angles does not result in equal linear segments. See text for details.
How Sound Points
Acoustic wavelengths are not only longer for low frequencies and shorter for highs, but straighter for highs and more bendy for lows. If you wanted to “aim” a sound wave with great accuracy, a high frequency is a good choice because it tends to move in a relatively straight line. Wavelengths of low frequencies, on the other hand, can wrap around objects and, in general, be a curvy aural nuisance. The directional properties of sound waves are one of the many important variables engineers consider when they design warning sirens, such as those used on emergency vehicles or weather alert systems. The text has some additional information on the consequences of relatively long versus short wavelengths.
When the Linear Projection of Uniform Circular Speed Is Stretched Out in Time, the Result Is a Sine Wave
Imagine now that the rotating object in Figure 7–6A is a small ring through which a pencil is inserted and that the motion described by UCS is marked on paper beneath the circle. If the circle is moved from left to right across the paper at a constant speed and without disturbing the UCS of the rotating ring, the pencil line draws the motion as a function of time. The resulting waveform would look like the one on the right in Figure 7–7 (and the ones in Figures 7–3 and 7–5) and is called a sine wave. This is displacement shown as a function of time. If the time scale is known, the period (and its inverse, frequency) can be determined using the simple computation of Formula (1)—f = 1/T. Note also the corresponding positions on the circle and resulting waveform (given by A, B, C, D in Figure 7–7).
Figure 7–7. Linear projection of uniform circular speed, shown as a function of time. If a pencil is inserted through the ring at A on the large circle (left side of figure) during uniform circular speed, and the motion is traced as the pencil is moved from left to right, the result is the sinusoidal waveform on the right side of the figure. Angular notation on the waveform at the right corresponds to angles crossed during motion around the circle on the left.
Sinusoidal Motion Can Be Described by a Simple Formula and Has Three Important Characteristics: Frequency, Amplitude, and Phase
Sinusoidal motion can be described by three characteristics, or parameters. The first of these parameters is frequency, which has been defined above as the number of full cycles occurring in a 1-s interval. If one complete rotation about a circle is equivalent to one cycle of vibration, it is easy to see how a faster speed of rotation results in a higher frequency. A faster speed of rotation reduces the amount of time required to complete one full revolution around the circle. This is equivalent to saying that the period, T, is reduced with faster circular speeds. As Formula (1), f = 1/T, and Figure 7–4 indicate, a reduction in T is associated with an increase in f.
The second parameter is amplitude, symbolized as A. Amplitude can be thought of in terms of displacement of an air molecule from the rest position. The circle in Figure 7–6 provides a simple illustration of variations in A. The vertical line onto which points are projected from the circular motion corresponds in length to the size (diameter) of the circle. The extremes of the linearly projected motion are, therefore, defined by the diameter of the circle. If the circle is made larger or smaller, the extremes of the linearly projected motion are greater or smaller, respectively. Amplitude of vibration (for the current purposes, the same as molecule displacement) is directly related to the size of the circle from which sinusoidal motion is derived.
The third parameter, phase, symbolized by the Greek letter φ (phi), describes the position of the sinusoidal motion relative to some arbitrary reference position. For example, in Figure 7–7, assume that position A is the reference point. Using the circular basis of sinusoidal motion, the location or phase of point B can be described as 90 degrees relative to point A, because the radii extending to points A and B form a 90-degree angle. Similarly, the phase of points C and D are 180 degrees and 270 degrees, respectively, relative to the reference point A. This phase angle description applies to the waveform in Figure 7–7 as well, because points along the waveform are simply points around the circle, extended in time. With A as the waveform reference point, a time corresponding to a lag of 90 degrees is equivalent to a quarter of a complete cycle, 180 degrees would be a half cycle, and so forth.
These examples use phase to compare points within a single waveform, but phase can also be used to compare points across two waveforms of identical or different frequencies. Phase relations between two or more sinusoids of different frequencies are discussed below in the section dealing with complex waveforms.
A simple formula describes sinusoidal motion as a function of time. This sinusoidal function is used to determine the displacement of an object (i.e., an air molecule) at any instant in time, and is computed as follows:
Tables of sine values for angles between 0 and 360 degrees are available in any basic trigonometry text or on the Internet. Sine values vary between 0 and +1.00 for angles ranging from 0 to 90 degrees, and between +1.00 and 0 for angles ranging from 91 to 180 degrees. Between 180 and 360 degrees, these values have mirror-image negative values, ranging from 0 to −1.00 (270 degrees) and then back to 0 (360 degrees).
If the starting phase (φ) in Formula (3) is assumed to be zero (that is, if we are not concerned about the absolute starting phase of the waveform), the formula for sinusoidal motion can be simplified to:
where D = displacement, A = maximum displacement, as above, and θ = the angle formed between the reference radius (i.e., the radii projected to point A in Figures 7–6 and 7–7) and the radius projected to any other point along the circle. For the sequence of angles formed as an object rotates around the circle (that is, 0 to 360 degrees), application of this formula yields a sine wave.
A sine wave is a waveform that results from the linear projection of UCS. A sine wave is periodic, because multiple rotations around the circle produce a sequence of multiple waveforms having the same period. Because the period is the same for all cycles of the vibration, sinusoids by definition and derivation have only a single frequency. A sinusoidal waveform can be described by a simple formula in which period, amplitude, and phase are the parameters.
Because a sinusoid involves only a single frequency, it is the simplest type of acoustic event. Sinusoids are the “building blocks” of acoustic events containing many frequencies. Acoustic events that contain many frequencies have complex waveforms, which may or may not repeat over time. The next section describes complex acoustic events.
Two types of complex events are considered: (a) those in which the waveform pattern repeats over time, and (b) those in which no repeating pattern can be identified. When an acoustic event contains more than one frequency and has a waveform with a repeating pattern, it is called a complex periodic event. An acoustic event with more than one frequency and no repeating pattern is called a complex aperiodic event.
Complex Periodic Events Have Waveforms That Repeat Their Patterns Over Time and Are Composed of Harmonically Related Frequency Components
Figure 7–8 shows two different complex periodic waveforms and associated spectra. Both waveforms, shown above their respective spectra, share the property of a repeating pattern over time and have the same period of roughly 8 ms and hence a fundamental frequency of around 125 Hz (f = 1/8 ms = 1/.008 s = 125 Hz). The fundamental frequency is determined by the rate of repetition of the major waveform pattern, as indicated by the marked periods on the two waveforms. Even though the fundamental frequency is nearly identical for the two waveforms, the appearance of the waveforms is clearly different. The frequencies in addition to the fundamental frequency, and their amplitudes and phase relations, give the waveforms their unique appearance.
Waveform displays are said to show an acoustic event in the time domain, because the event is shown as a function of time. This is a useful way to display an acoustic event, but if one is interested in the frequencies and their amplitudes that give waveforms their unique appearance, a different type of display is required. When an acoustic event is examined in the frequency domain, the individual frequencies and amplitudes that contribute to a complex acoustic event are displayed. The frequency domain of an acoustic event is shown by a spectrum, which can be defined as a plot of relative amplitude (y-axis) as a function of frequency (x-axis). Spectra are shown immediately below the two waveforms in Figure 7–8; the two spectra are quite different.
The spectrum of the waveform in Figure 7–8A shows frequency components at odd number multiples (1, 3, 5, 7, 9 . . . n) of the fundamental frequency (125 Hz). This spectrum contains frequency components at 125 Hz: the fundamental frequency, or first harmonic (H1), 375 Hz (H3), 625 Hz (H5) . . . 125 × (2n − 1) Hz, where n is the number of harmonic components in the spectrum. The relative amplitudes of the frequencies, represented by the heights of the harmonic components (i.e., the magnitude on the y-axis), decrease consistently as frequency increases. In this spectrum, therefore, a higher frequency (e.g., 625 Hz, the fifth harmonic of 125 Hz) always has less energy than a lower frequency (e.g., 375 Hz, the third harmonic).4 Waveform (A) is a common test signal used in electronics shops and laboratories, and is called a triangular wave. A triangular wave is said to have an odd-integer series of harmonics.
Figure 7–8. Two complex periodic waveforms and their spectra. A. Waveform and spectrum of a 125-Hz triangular wave. The period of one cycle (8 ms) is marked on the waveform, and the first (H1), third (H3), and fifth (H5) harmonics are marked on the spectrum. B. Waveform and spectrum of the vowel /a/ produced by an adult male. The period of one cycle (7.99 ms) is marked on the waveform, and the first (H1), second (H2), and third (H3) harmonics are marked on the spectrum.
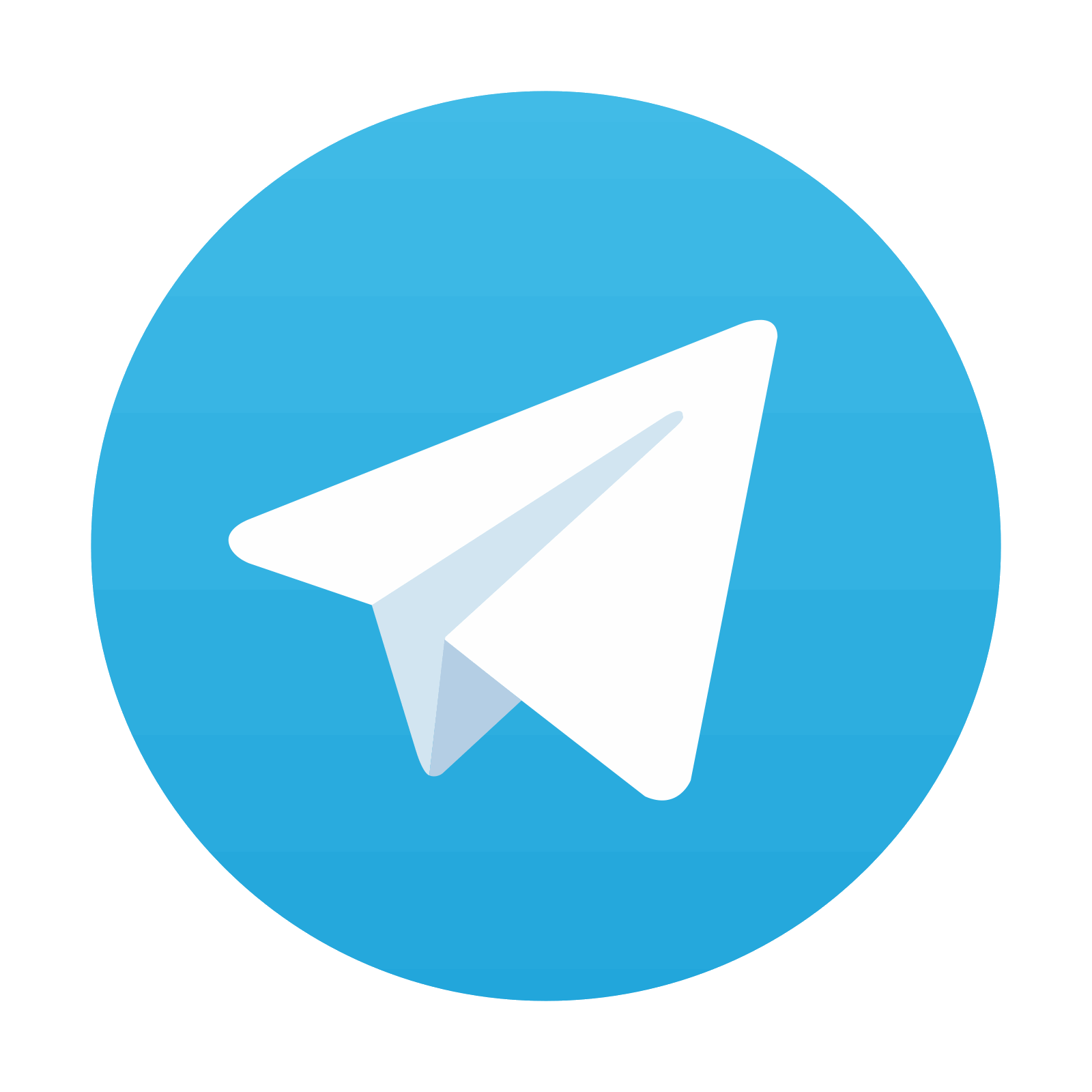
Stay updated, free articles. Join our Telegram channel
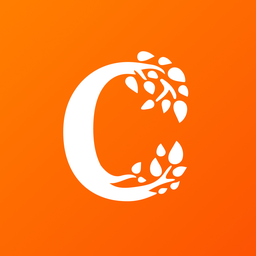
Full access? Get Clinical Tree
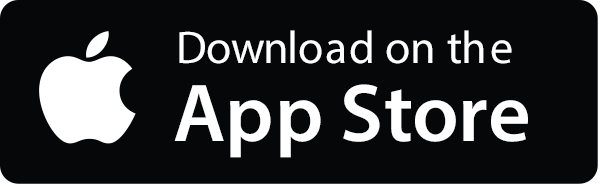
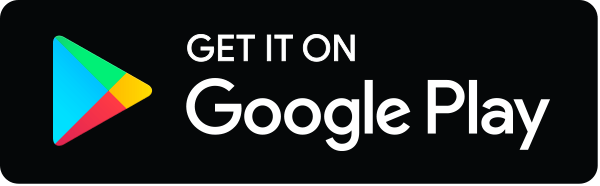