FIGURE 7.1 A montage of a whole mounted human fetal retina at 26 WG, immunolabeled to show rod photoreceptors (green) and retinal blood vessels (red) and imaged by confocal microscopy. Developing retinal vessels approach the incipient fovea from the nasal side (left) as well as above and below. The FAZ is defined when the superior temporal and inferior temporal quadrantic arteries (above and below, respectively) meet along the raphe in temporal retina sometime between 25 and 28 WG, approximately.
The FAZ appears to be specified by local expression of the antiangiogenic pigment epithelium–derived factor (PEDF) by ganglion cells at the incipient fovea and in the emerging fovea during its formation (23,24). Those studies also find that EphA6 (a receptor for the axon guidance factors ephrin-A1 and ephrin-A4) may play a role in vascular patterning around the FAZ and likely accounts for the slow growth of vessels into the posterior pole of the retina (25). EphA6 is also highly expressed by ganglion cells at the incipient and developing fovea, and in other animals EphA6 has been found to regulate mapping of ganglion cell axons into the appropriate areas of the visual target nuclei in the brain. The EphA6 molecule interacts with its ligands, ephrin-A1 and ephrin-A4, in a repellant response—such that cells expressing the ligand are turned away from areas of high receptor expression. Studies show that in the retina, astrocytes (which lead retinal endothelial cells in their migration across the retina) express the ephrin-A1 and ephrin-A4 ligands. This finding indicates that astrocytes, and hence retinal vessels, tend to be repelled from the incipient foveal region where EphA6 is highly expressed, resulting in the slow growth of retinal vessels into the foveal region during development (25).
Development of the retinal vessels results in a retina that is scaffolded by a capillary plexus throughout, except at the FAZ. It is proposed that as a result, the FAZ is more responsive to mechanical forces acting on the retina. That is, because the FAZ is avascular, it is more deformable and more elastic than the rest of the vascularized retina (26). Using this observation as a premise, Springer (14,15) has used finite element analysis to model the effects of pressure (resulting from intraocular pressure) and stretch (resulting from growth of the eye) on formation of a fovea. The findings of modeling, combined with careful observation of the growth surges in the posterior eye, suggest that intraocular pressure acts on the retina within the FAZ to initiate formation of the foveal depression, soon after definition of the FAZ. By closely matching changes in the fovea with analysis of retinal growth, the findings also suggest that when the retina enters a period of very rapid growth after birth, the FAZ and the early fovea are affected by growth-induced stretch, which modifies the profile of the fovea making it more shallow, and the sides more gently sloping, than in the intrauterine period (26). Previously it has been proposed that tension on ganglion cell axons during development, like that which would result from retinal stretch, may be the mechanism that mediates centrifugal displacement of ganglion cells that results in excavation of the fovea, during the late prenatal and postnatal phase of development (27).
DIFFERENTIATION OF CONES: NORMAL DEVELOPMENT
The maturation of the cone photoreceptor population during intrauterine and early postnatal development follows a rather surprising trajectory. That is, even though foveal cone photoreceptors are the first cells to differentiate in the retina, they are the last to achieve the adult-like characteristics of foveal cones. This oddity in maturation of foveal cones has been known of for at least a century (6) but is now achieving clinical significance, since the delayed maturation of the fovea is now widely recognized as a feature that distinguishes OCT images from adult and neonatal maculae (28–31). The differences between foveal cones and those on the foveal rim are illustrated at midgestation (20 WG) and full term (40 WG) in Figure 7.2. At 20 WG cones in the foveal cone mosaic (“fovea”) are similar in size and appearance to their neighbors on the foveal rim, which are interspersed with rods. At 40 WG, cones in the central fovea are only a little more differentiated and slightly taller than at 20 WG. In contrast, cones on the rim of the fovea at 40 WG are highly differentiated and significantly narrower and taller than those on the rim at 20 WG or within the fovea at 40 WG. Thus, the fovea is immature at birth in two ways. First, the outer nuclear layer (ONL) comprises cone photoreceptors of a very immature morphology, which are not stacked into tiers of cells (as in the adult fovea); rather, they remain in the original monolayer of cuboidal cells and are little changed in shape on size since midgestation. Second (as we see below), at full term the fovea still includes cells in the ganglion cell and inner nuclear layers (INLs).
FIGURE 7.2 Cones from within the fovea, and from the foveal rim, drawn to scale at midgestation (20 WG) and at full term (40 WG). At 20 WG cones are cuboidal cells apposed to the RPE layer. They have no axonal process, and the inner and outer segments are undifferentiated, as seen by light microscopy. Cones on the foveal rim at 20 WG are beginning to elaborate axonal processes, and differentiation of the inner and outer segments is slightly more advanced. At 40 WG cones on the foveal rim are adult-like, having distinct axonal processes that are sandwiched by rod somas, as well as distinct inner and outer segments. However, in the fovea at 40 WG cones are relatively unchanged since midgestation; inner and outer segments are becoming evident, and the axonal processes are beginning to narrow. However, overall they are only slightly taller than cones at 20 WG and significantly shorter than those on the foveal rim, at birth.
The cellular mechanisms that mediate morphologic transformation of cones are not clearly understood. It is known that aggregation and elongation of photoreceptors in central retina is common among primates, even in those species that do not have a fovea (32). Narrowing and elongation of cones is the key mechanism that mediates the accumulation of cones in the fovea and adjacent macula (33), a process commonly referred to as “cone packing.” By becoming narrower and more elongated, cones can be packed tightly together in a space-efficient hexagonal pattern, allowing more cones to be accommodated per unit area in the foveal cone mosaic, while the cell–cell relationships that were established early in development are preserved. Thus, changes in cell shape mediate cone packing. Some data suggest that fibroblast growth factor (FGF) 2 signaling via FGF receptors, which are differentially distributed across the soma, axon, and inner and outer segments of cones during development, mediates these changes in cell shape (34,35).
Establishment of a high density of cones in the foveal cone mosaic is the anatomical basis of high-acuity vision, and foveal acuity is directly proportional to the packing density of cones within the foveal cone mosaic. Developmentally (and evolutionarily) there is a drive toward increased cone density in the retina in the foveal cone mosaic. The spatial density of cones in the foveal cone mosaic increases from around 10 k/mm2 to approximately 30 k/mm2 during intrauterine life (2,33), without newly generated cells being added to the mosaic. This increase in cone density would be seen to be even greater if cone density in the parafovea (rather than fovea) was compared over time, since it is now understood that maturation (narrowing and elongation) of cones in the fovea, and hence their packing, is delayed until the postnatal period, while cones adjacent to the fovea achieve adult-like features prenatally (6,34,36–38).
The spatiotemporal pattern of cone packing over time can be observed in flat-mounted retinas, using the population of cones sensitive to blue/short wavelength light (S-cones) as a population “marker.” S-cones comprise approximately 6.8% of the cone population within 4 mm of the foveal center (39). They can be identified in postmortem retinas using an antibody against the short wavelength-sensitive opsin contained in the outer segments of mature photoreceptors and also present in the cell bodes of developing S-cones (4). In Figure 7.3, the spatial density of S-cones along the horizontal meridian of the retina, between the fovea and optic disc, is shown at three different ages—18 WG, 6 weeks postnatal (P 6 wks), and in the adult (data from [40/h]). The graphs show that S-cone density just outside the central fovea (at ~15% of the fovea-to-optic disc [OD] distance or ~500 μm eccentricity) increases between 18 WG (pale blue line) and adulthood (black line), while S-cone density near the optic disc is significantly reduced over the same period. The graphs indicate that high cone density near the fovea, and in the macula in general, is achieved by mass displacement of cones from more eccentric locations (centripetal displacement, indicated by the arrow), since there is no detectable cell death during this period, and no photoreceptors are added to the mosaic (4). Note that the S-cone population cannot be used to indicate changes in total photoreceptor density closer than approximately 1 mm from the foveal center, because S-cones are absent from the fovea in adults and throughout development.
FIGURE 7.3 Graphs illustrating the change in distribution of cones in the optic discto- fovea region, between 18 WG and adulthood. The population of cones sensitive to blue light (short wavelength-sensitive or S-cones) has been used as a marker population, representing approximately 6.8% of the total cone population. No photoreceptors are added to the mosaic in this region after approximately 18 WG, and there is negligible cell death. The graphs show that early in development, cones are more numerous near the disc than near the fovea, but during in utero development, there is mass displacement of cones toward the fovea (indicated by the arrow). This displacement continues for some months, postnatal. Thus, the mechanisms that affect displacement of cones toward the fovea act over quite long distances—at least up to approximately 3 mm. Note that because S-cones are absent from the fovea, S-cone numbers in the 10% closest to the fovea (left side of the figure) are not indicative of the total cone population. (From Cornish E, Hendrickson A, Provis J. Distribution of short wavelength sensitive cones in human fetal and postnatal retina: early development of spatial order and density profiles. Vision Res 2004;44:2019–2026.)
These data, along with morphologic observations of cone development, suggest that displacement of photoreceptors is achieved as a result of a wave of photoreceptor elongation, which passes from the disc toward the fovea, over a period of several months (34,38). In humans and macaques, the passage of this wave coincides approximately with the spread of the outer plexus of the retinal blood supply toward the fovea. Thus, the wave of cone maturation may be attributable to the delivery of additional oxygen and metabolites by the newly formed deep retinal plexuses. Because increased oxygen supply allows for an increase in metabolic rate, it is conceivable that the wave of accelerated development of these cells is driven by this new blood supply. Although the literature is somewhat confused on the subject of formation of the deep retinal plexuses in the perifoveal region (9,41,42), the study by Provis et al. (9) clearly shows cross-sectional images of the developing fovea in the fetal macaque, evidencing that (i) the innermost layer of vessels defines the FAZ, (ii) the deep capillary plexuses first form in the vicinity of the optic disc, and (iii) the deep capillary plexuses around the fovea form just before birth and do not anastomose with the innermost layer of vessels until the early postnatal period (see Provis 2001, Fig. 11). The completion of the perifoveal anastomosis is consistent with expression of antiangiogenic factors, which retard endothelial cell proliferation, and hence the formation of anastomotic branches around the fovea (23–25). Postnatal development of the deep plexus surrounding the fovea coincides with the maturation of foveal cones (in the postnatal period), reinforcing the idea that provision of additional metabolites is important in the final phase of cone maturation.
The significance of these observations is that where normal development of the retinal blood supply is interrupted— for instance, by prematurity—there are clear implications for neural development.
One of the major consequences of premature delivery/low birth weight may be reduced expression of vascular endothelial growth factor (VEGF) in the retina, resulting from exposure to higher levels of oxygen than would normally occur in utero. A degree of hypoxia in the retina is normal and drives development of retinal vessels (43). Under in utero conditions the unvascularized regions of the retina (~25% retinal surface area at 24 WG) express high levels of VEGF under influence of hypoxia-inducible factor, promoting division and migration of endothelial cells. This hypoxia is reduced when infants are placed in oxygen-rich environments, resulting in slowing or inhibition of further vascularization of the retina. When babies are returned to room air, reduced oxygen availability can promote expression of VEGF and trigger an overgrowth of retinal vessels. To understand the impact of prematurity on foveal development, we need first to consider the developmental processes that are active, particularly in central retina, at a few relevant stages: 24 WG, 28 WG, and 32 WG.
At 24 WG approximately 50% of the retina is still engaged in neurogenesis, but not in the region around the posterior pole, including the emerging fovea (44). The retinal vasculature covers approximately 75% of the surface area of the retina, but in most examples that have been examined, retinal vessels are just approaching the (incipient) foveal region and the FAZ is wide open on the temporal side (see Fig. 7.1) (41,44); see however (42). The foveal depression has not begun to form. Some of the most vulnerable infants are born around 24 WG, with increasing numbers of these infants surviving in the modern intensive care nursery environment. This group includes many with a birth weight <1,000 g, who are three times more likely than those born at full term to have acuity <6/60 and experience a range of visual impairments—including strabismus, amblyopia, and color vision defects—suggestive of anomalies of both the macula and central visual pathways (11,45,46).
At 28 WG the FAZ is defined by the innermost vascular plexus, at the GCL/inner plexiform layer interface, but there are no vessels present in the deeper layers of the retina around the fovea (41). The human retina at 28 WG is approximately equivalent to the macaque retina at 130 days postconception (dPC), in which more specimens have been studied. At this age the foveal depression is prominent, although all of the retinal layers are still present. There is no evidence of a deep plexus in the immediate vicinity of the fovea (9), although vessels are present on the inner and outer aspects of the INL, within a millimeter or so of the fovea. These features of the macaque retina at 130 dPC are consistent with the drawings of Bach and Seefelder (6) of a human fetus at 28 WG. In both cases, the foveal and perifoveal cones are cuboidal cells showing little evidence of inner and outer segment formation, or elaboration of their axonal processes.
By 32 WG vessels are present in the deeper plexuses within about 200 μm of the FAZ margin, although anastomoses between the different layers are not detected within about 500 μm of the FAZ margin. These vessels grow very slowly toward the FAZ margin—most likely under the control of antiangiogenic factors expressed during this period—and do not form the anastomoses that will complete development of the perifoveal capillary bed until the early postnatal period. Cones of the perifovea and on the foveal rim are distinctly elongated at 32 WG, although those in the foveal center remain cuboidal with rudimentary inner segments evident in histologic sections.
At least two studies now report that the FAZ is significantly smaller in people born prematurely (29,47). Direct comparison of the data from those two studies is not possible owing to the different approaches used and differences in the gestational age range selected to define prematurity. Despite this, the findings of each indicate that the diameter of the FAZ is reduced by at least 50% in premature infants. There is no simple explanation of this observation. Our present understanding is that antiangiogenic, as well as guidance, factors regulate vascular patterning around the fovea and define the FAZ. We also know that retinal vascular growth is delayed by exposure to an oxygen-enriched environment. Thus, in premature infants who spend several days or weeks of the early postnatal period in a humidicrib, the formation of the perifoveal plexus is likely to be delayed by an equivalent number of days or weeks. Based on our current understanding, there are at least two possible mechanisms in effect. First, premature birth and related environmental factors may directly modify expression of the antiangiogenic factors that define the FAZ. Second, the later timing of plexus formation around the fovea (as a result of time spent in an oxygen-enriched environment) may result in the FAZ forming at a later stage (e.g., 30 WG rather than 28 WG), when antiangiogenic and guidance factors may be in natural decline, allowing the capillaries to “overgrow.”
Several studies now report that prematurity is associated with a more shallow foveal depression and a thicker central fovea (21,29,48–51). Increased retinal thickness at the fovea in individuals born prematurely is attributable to two factors. First, persistence of the GCL and INL in the central fovea suggests reduced migration of inner retinal neurons out of the fovea in these cases Second, OCT indicates that individuals born prematurely have increased thickness of the layer comprising photoreceptor cell bodies (ONL) and their axons (Henle fibers)—suggestive of greater elongation, and possibly packing, of cones in the central fovea. Some caution should be exercised with this latter interpretation, because of the complex composition of this layer as seen in OCT and also because foveal morphology is influenced by a variety of factors including gender and race (52,53), which complicate evaluation of OCT images. However, the findings do indicate that the mechanisms that mediate centrifugal migration of inner retinal cells resulting in the formation of a foveal depression on the inner retinal surface are independent of the mechanisms active in the outer retina, which mediate centripetal displacement of cones and hence cone packing.
Further investigations that take morphogenetic variations into account, and which correlate FAZ diameter with both (i) time spent in oxygen enrichment and (ii) with gestational age at birth, are needed to more fully explore and understand the variables that contribute to FAZ size and shape. Broadly, we can predict that because by 28 WG the FAZ is already defined, and the fovea is in the early stages of development, differences in FAZ size and foveal morphology in infants born after this time point would be less pronounced than in those born earlier. Indeed, two studies have now reported that retinal thickness at the fovea is correlated with gestational age (50,54), including an analysis indicating that 28.9 weeks is a “critical gestational age” for premature birth in terms of impact on fine foveal structure (50).
While analysis of foveal morphology in infants born prematurely reveals distinct changes associated with premature birth, functional evaluations of vision suggest that the defects often associated with prematurity are not well correlated with macular or foveal morphology. In particular, a poorly developed or absent fovea in an infant born prematurely or at very low birth weight does not necessarily correlate with poor visual acuity (29,47,50,54). Some data suggest that premature birth is associated with changes in photoreceptor responses and other subtle neurovascular anomalies (55). However, relatively little attention has been paid to the effects that modification of the retinal environment at critical stages of development—as occur in birth before 32 WG—may have on the target visual centers in the brain. As noted in the preceding section, premature birth modifies the expression profile of key morphogenetic factors in the brain, the best known of these factors being VEGF, which influences endothelial cell proliferation and migration, and underlies the pathogenesis of ROP. We also hypothesized that expression of the factors that regulate vascular growth in the macula and define the FAZ, including PEDF and EphA6, is modified by premature birth—indicated by the smaller size of the FAZ in individuals born prematurely.
Expression of both VEGF and PEDF is modulated by oxygen (56,57), and up-regulation of Eph receptor signaling pathways has also been identified in models of oxygen-induced retinopathy (58). We have previously identified high levels of expression of EphA6 in ganglion cells at the incipient fovea in both fetal human and macaque retinas (24) and found that expression of EphA6 at the fovea intensifies and becomes more localized at the fovea after definition of the FAZ (25). Eph/ ephrin signaling has a key role in the mapping of retinal projections in small mammals (59–61). In humans, EphA signaling also participates in mapping the projection of the retina onto the dorsal lateral geniculate nucleus (62), which commences in utero and continues into the postnatal phase of development (63). It is reasonable, therefore, to conjecture that expression of EphA6 by ganglion cells in the immediate vicinity of the fovea makes a significant contribution to retino-geniculate mapping, both pre- and postnatal in humans, and that signaling in this pathway is modified by premature birth. Thus, the earlier the birth, the greater would be the disturbance to the cell-signaling mechanisms that mediate mapping in the brain, resulting in poorer visual outcomes for those individuals. While these mechanisms remain largely hypothetical at present, the rapid progress of tensor diffusion imaging (TDI) means that it will soon be possible to visualize major brain tracts and evaluate the effects of prematurity in the brain, including visual pathways (57,64–66). A key element of analyses of visual pathways will be evaluation of central retinal development by OCT, including FAZ diameter and foveal morphology, in which deviations from the norm will serve as correlates of downstream modifications of brain structure, identified by TDI.
WHAT CAN WE LEARN FROM PERINATAL OCT?
The typical features of a normal full-term retina are shown in Figure 7.4, which comprises an OCT image along with a photomicrograph scaled to mimic the aspect ratio (x:y = 1:4.3) of the OCT image (Fig. 7.4A) and the same histologic section shown at full resolution (Fig. 7.4B). Typically, OCT scans 6 to 9 mm of retina centered on the fovea—a considerably more extensive region of retina than is usually observed in photomicrographs. The specimen shown in Figure 7.4B comprises three adjacent fields of view imaged using a 20x objective lens and montaged to show 2.4 mm of retina centered on the fovea, compared with 6.3 mm of retina represented by the OCT image (Fig. 7.4A). Within the fovea of a fullterm retina, the GCL, inner plexiform, and INLs are still present. Ganglion cells and bipolar cells complete their centrifugal migrations out of the fovea during the first several weeks of postnatal life, so that in the adult retina, an accumulation of cone photoreceptor cell bodies lines the fovea—save for a few ganglion cells that might be present in a normal adult fovea. Another prominent feature at the fovea is the presence of a single band of hyperreflectivity at the level of the retinal pigment epithelium (RPE), compared with multiple hyperreflective bands toward the edge of the image (2.5 to 3.0 mm eccentricity). This difference reflects the relatively immature state of foveal cones at birth (see also Fig. 7.2).
FIGURE 7.4 Sections through the fovea at 40 WG using OCT and by histology. A: The layers of the retina are labeled using conventional terminology (left), and the hyperreflective bands 0 to 4 are indicated to the right. Band 0 corresponds to the layer of cone pedicles (the outer plexiform layer), which is continuous through the fovea in the newborn retina, but absent in the adult due to the outward displacement of bipolar cells from the fovea. Bands 1 and 2 are distinct about 1.5 mm or more from the central fovea; band 1 represents the ELM and band 2 the more dense part of the inner segment, the ellipsoid. Bands 3 and 4 each represent components of the RPE; they are seen separately in the adult fovea, but not at 40 WG because the photoreceptors are not fully elongated. The key shows the foveal region of a 40 WG, stained with H&E and scaled to the same aspect ratio used for the OCT (1x: 4.3y). B: The same section enlarged to show cellular details. Note the elongation of cones and Henle fibers on the foveal rim (arrowheads), compared to the central fovea where the cones are neither elongated nor packed. GCL, ganglion cell layer; HFL, Henle fiber layer; INL, inner nuclear layer; IPL, inner plexiform layer; NFL, nerve fiber layer; ONL, outer nuclear layer; OPL, outer plexiform layer. (OCT image by courtesy of Adam Dubis and Joseph Carroll.)
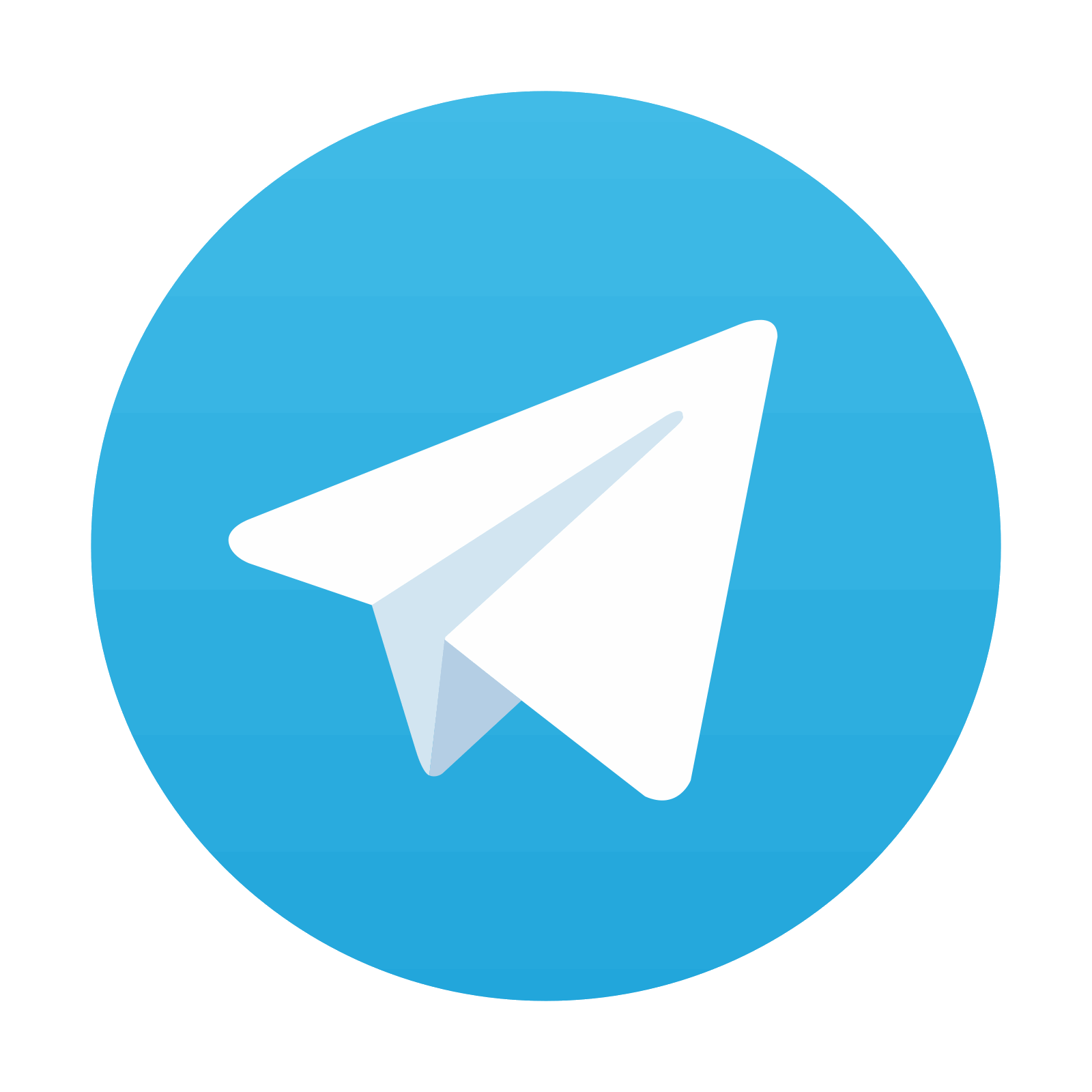
Stay updated, free articles. Join our Telegram channel
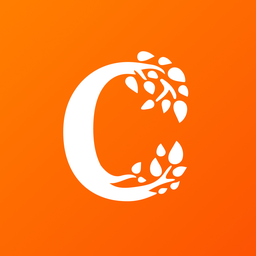
Full access? Get Clinical Tree
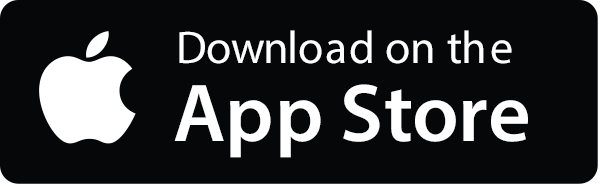
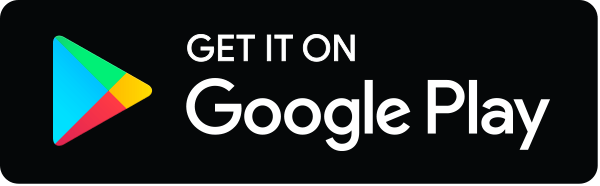