FIGURE 42.1 Comparison of mouse (A) and rat (B) OIR following oxygen exposure and return to room air. The pattern of pathology in the rat more accurately mimics the human pattern (C). (C: Reprinted from Lepore D, Molle F, Pagliara MM, et al. Atlas of fluorescein angiographic findings in eyes undergoing laser for retinopathy of prematurity. Ophthalmology 2011;118:168–175, with permission.)
Despite the early and continuing utility of these models, there remained a critical need for animal models that both reflected the blood oxygen concentrations experienced by preterm infants in modern-day NICUs and promoted a human-like pattern of vessel growth retardation, creating a peripheral avascular region. The most widely used model that accomplishes this is the variable oxygen rat model developed by Penn et al. in 1993. Compromised lung function and variability in oxygen delivery resulting from clinical interventions cause significant fluctuations in arterial blood oxygen partial pressure, PaO2, in preterm infants (40–42). In order to mimic these fluctuations, Penn et al. (43) exposed rat pups to fluctuating levels of oxygen, followed by a postexposure period in room air. During exposure, rat pups experienced oxygen concentrations that cycled between 80% and 40% every 12 hours. This variable oxygen model induced preretinal neovascularization (NV) in 66% of oxygen-exposed pups, whereas exposure to constant 80% oxygen rarely (13%) resulted in preretinal NV after room air postexposure. Penn et al. (44) subsequently improved the clinical relevance of the model by employing alternating hyperoxic and hypoxic episodes to reproduce PaO2 values and fluctuations in rats that mimicked those experienced by premature infants in whom ROP develops. Findings indicated that the variability in PaO2 was the most critical variable in determining OIR outcome (44). Thus, the benefit of the rat model is its production of relevant, fluctuating PaO2 levels resulting in a human-like pattern of retinal vascular pathology. In the rat, as in the human, the resulting NV develops at the boundary between vascular and avascular zones, and the NV severity is directly correlated with the extent of retinal avascularity at the time of removal to room air. A comparison of the vascular patterns in mouse and rat is shown in Figure 42.1.
FIGURE 42.2 The effect of O2 saturation range on survival, cerebral palsy, and the development of threshold ROP. (Adapted from Tin W, Milligan DW, Pennefather P, et al. Pulse oximetry, severe retinopathy, and outcome at one year in babies of less than 28 weeks gestation. Arch Dis Child Fetal Neonatal Ed 2001;84:F106–F110.)
In addition to expanding our understanding of the mechanisms and factors that contribute to the development of ROP, animal models have also played a critical role in the early development of therapeutic interventions for the disease. Based on the early notion that supplemental oxygen leads to vessel attenuation through destruction of capillary endothelial cells by reactive forms of molecular oxygen, the potential efficacy of antioxidants became a popular subject of study for investigators. For decades the kitten model was used in studies of the efficacy of antioxidant therapy and specifically vitamin E supplementation, for prevention of ROP progression. In the mid-1970s, Phelps and Rosenbaum hypothesized that vessel closure during hyperoxemia was due to damage from lipid-soluble oxygen free radicals. Therefore, they administered alpha-tocopherol, a free radical scavenger, to kittens exposed to constant hyperoxia (~80%) for 2 to 3 days. In this study, alpha-tocopherol significantly reduced vessel attenuation, and this suggested that vitamin E might be efficacious in the treatment of ROP (45). Later studies expanded the use of antioxidant therapies to other models of OIR. Penn et al. (46) exposed newborn albino rats to 60% oxygen for 14 days to determine the effect of oxygen exposure on retinal levels of antioxidants. They found that retinal alpha-tocopherol and ascorbic acid were reduced, while glutathione reductase, S-transferase, and peroxidase were unchanged compared to room air–raised controls (46). Therefore, the investigators administered vitamin C and E through dietary manipulation of the mothers to raise the nursing pups’ retinal ascorbic acid and alpha-tocopherol levels, respectively. Vitamin C supplementation of mothers showed no effect on vasoattenuation in oxygen-exposed pups. Conversely, vitamin E dietary intake in mothers throughout gestation and the 14-day oxygen exposure increased retinal alpha-tocopherol three- to fourfold in oxygen-exposed pups, significantly reduced avascular retinal area, and increased retinal vessel density (46).
Spierer et al. (47) overexpressed the naturally occurring enzyme copper–zinc superoxide dismutase (CuZn-SOD) in mice and exposed the animals to OIR conditions. Overexpression of the CuZnSOD antioxidant significantly reduced central vasoconstriction, vessel tortuosity, and preretinal NV compared to exposed wild-type animals (47). Transgenic mice also have been used to determine the potential pathogenic role of nitric oxide (NO), which is produced by endothelial nitric oxide synthase (eNOS). NO-derived peroxynitrite is a known mediator of oxidative injury in endothelial cells and is elevated in infants with bronchopulmonary dysplasia (48). Brooks et al. (49) demonstrated that eNOS-defficient mice or pharmacologic inhibition of the enzyme in the mouse OIR model both demonstrated protection by limiting oxidative stress. This study showed that eNOS specifically affected immature vessels, and the work stimulated further study of eNOS activation in other animal models of OIR. For example, in the rat model of OIR, eNOS activation significantly increased vessel tortuosity, a common feature of human plus disease (49).
Effects of Oxygen on Phase 2 ROP: Vasoproliferation
In the mid-1980s, Phelps and Rosenbaum (50) tested the idea that supplementation of oxygen in the latter stages of ROP could be useful for reducing vasoproliferation. They exposed 3-day-old kittens to hyperoxia for 65 hours and then allowed them to recover in one of three oxygen environments: room air (21%), a variable hyperoxic/hypoxic environment (~43% to 8%), or a tapered withdrawal of oxygen to room air. Kittens that were exposed to variable oxygen during the postexposure period had significantly less severe retinopathy than those exposed to constant room air conditions. The result of gradual withdrawal from hyperoxia was not significantly different from that of direct removal to room air (50). This early study demonstrated that the oxygen conditions experienced by the newborn during recovery from an acute oxygen-induced injury are critical to the healing process and laid the foundation for future studies on the effects of supplemental oxygen in ROP.
The rat model also has proven to be useful in determining the effect of supplemental oxygen manipulation in the care of premature infants. The model has been used by several investigators to determine the possible benefit of using supplemental oxygen therapy for ROP recovery (51,52). Following exposure to the 50/10% variable oxygen paradigm, rat pups were returned to room air or a supplemental ambient oxygen environment (28%) for 4 days. While VEGF levels were reduced with supplemental oxygen treatment, no difference in NV severity was observed. This conclusion opposed early findings from the kitten model and was a more accurate predictor of the outcome of supplemental oxygen clinical trials, which will be discussed in the next section.
Fluctuating blood arterial oxygen levels in the rat model of OIR were also critical to our understanding of the molecular mechanisms involved in ROP pathogenesis. The extended bouts of hyperoxemia and hypoxemia imposed on the newborn rats, also experienced by sick preterm infants, dramatically affect the levels of angiogenic and antiangiogenic factors in the developing eye. This is particularly true of VEGF, which is directly implicated in both vasoattenuative and vasoproliferative tissue responses. Werdich et al. (53) demonstrated the effect of inspired oxygen fluctuations on retinal VEGF production by showing that hyperoxemia contributes to the delay in vascular growth toward the periphery by reducing VEGF production and that subsequent NV can be attributed to excessive production of VEGF triggered by the large avascular zones during room air postexposure. Evidence points to a sequestering of retinal VEGF, since its levels slowly climbed over the repeated hypoxic and hyperoxic tissue insults (53).
TARGETING OXYGEN IN THE CLINIC
Current Treatment Strategies
Based on data derived from animal models of OIR, some clinical trials have aimed to address phase 1 ROP by attempting to reduce vasoattenuation or mitigate its effects. It is reasoned that this would decrease retinal hypoxia and consequent excessive growth factor production and release, thereby slowing or preventing ROP progression. Currently, surgical intervention is the only approved treatment for advanced stages (stage 4 and 5 ROP), although anti-VEGF therapies have provided promising results in early trials (54). This section will focus specifically on the oxygen stress–related functions of surgical and anti-VEGF therapies.
Surgical intervention, predominantly in the forms of cryo- or laser ablation, remains the standard of care for stage 3+ ROP (10,54). Both cryotherapy and laser therapy are believed to act by a combination of mechanisms: by ablating oxygen-utilizing neurons, leading to better tissue oxygenation and lower growth factor production, and by destroying the cells that produce VEGF in response to tissue hypoxia—the Müller cells. Under normal circumstances, oxygen and nutrients diffuse from the choriocapillaris into the retina where the oxygen is entirely consumed by the photoreceptors, which have a very high density of mitochondria and high oxygen consumption (55). In retinal scars left by laser ablation, destroyed photoreceptors are partly replaced by gliosis of surviving Müller cells. Müller cells have few mitochondria and low consumption of oxygen. Hypothetically, this allows the laser scars to function as windows through which choroidal oxygen is free to diffuse into the inner retina, leading to increased oxygen tension. Oxygen tension in the inner retina following laser treatment has been studied in a number of experimental animals and in diabetic patients. The improved tissue oxygenation following retinal laser treatment was first shown in rhesus monkeys (56,57) and was confirmed in a number of subsequent studies (58–60).
The improved oxygenation of the inner retina and the relief of hypoxia lower the production of VEGF in the retina, and this is believed to be important in inhibiting NV, for example, in proliferative diabetic retinopathy. Application of panretinal photocoagulation therapy to patients with proliferative diabetic retinopathy alleviates oxygen stress. Aiello et al. (61) used vitreous samples from diabetic patients to determine the effect of photocoagulation treatment on VEGF release. Patients receiving laser photocoagulation therapy had reduced vitreous VEGF protein levels compared to patients with untreated proliferative diabetic retinopathy (61). Similarly, VEGF immunoreactivity is decreased following successful laser photocoagulation therapy, and this corresponds with a reduction in expression of VEGF receptors (62,63). Lip et al. (64) showed that serum VEGF levels were elevated in diabetics with proliferative retinopathy and subsequently fell following panretinal photocoagulation. These studies support the contention that improved oxygenation of the inner retina by laser therapy appears to effectively alleviate the NV response by reducing hypoxia-induced growth factor signaling.
In an attempt to reduce the NV of stage 3 ROP, the Bevacizumab Eliminates the Angiogenic Threat of Retinopathy of Prematurity trials employed a VEGF monoclonal antibody fragment, bevacizumab, previously approved for use in other ophthalmic applications. This strategy was designed to normalize upregulated retinal VEGF in the retinas of premature infants. A potential benefit to this therapy is that its use is independent of the oxygen environment, meaning that a clinician can provide oxygen in ranges that allow for decreased incidences of nonocular oxygen-related complications (10). Though scarring of the peripheral retina is spared, there are risks, including total retinal detachment, persistent avascular retina, later recurrence in disease, as well as potential risks associated with the intravitreal injection, including endophthalmitis (65). Premature infants may be particularly susceptible to the development of infection due to decreased capacity of defense mechanisms. Also, although anti-VEGF has been approved for the treatment of subretinal NV in the United States and has proven relatively safe in adults, the optimal dose, route of administration and type of anti-VEGF remains undefined for preterm infants (see also Chapter 39).
Oxidative Stress–Related Therapy
The retina is particularly susceptible to oxygen-mediated damage for several reasons: (i) Its cells have a high rate of oxygen consumption (66), even under normal oxygen conditions; (ii) it has the highest concentration of polyunsaturated fatty acids of any known tissue (67), and these molecules are preferred substrates for damaging lipid peroxidation reactions; and (iii) it is bathed by photons, and light is a known initiator of oxygen radical formation (68). Moreover, under therapeutic oxygen treatment, retinal PO2 is likely to be episodically elevated. The importance of oxygen-mediated damage to the retina also is suggested by the retina’s elaborate defense system. The retina can combat peroxidation by enzymatic detoxification of reactive oxygen molecules or termination of radical chain propagation by vitamin E. The rationale for using vitamin E as a treatment for ROP stems from the idea that peroxidative damage to cells is a causal factor and that, by acting as a scavenger of the reactive molecules that lead to peroxidation, vitamin E may prevent this damage. It is also important to note that reactive oxygen radicals can directly induce retinal VEGF production (69). Thus, antioxidant therapy may be beneficial for development of normal retinal vasculature and reducing the oxygen debt in the inner retina that can lead to NV.
It remains unclear whether, at premature birth, the retinal antioxidant system is sufficiently developed. It is known that premature infants are born with only 10% of the adult levels of retinal vitamin E (70). However, the tissue levels of other antioxidants (glutathione peroxidase, glutathione S-transferase, ascorbic acid) are substantially greater in premature infant retinas than in mature retinas (71). These comparisons reflect both vascular and avascular regions of the premature retina, compared with corresponding central and peripheral regions of the mature retina.
As discussed above, antioxidant therapy in the kitten and rat models proved efficacious in reducing vasoattenuation. Thus, human studies were performed in order to assess whether antioxidant supplementation could effectively prevent progression to threshold ROP. Vitamin E was the first biologic antioxidant agent introduced as a potentially suitable therapeutic for the treatment of ROP in 1949 (72). More extensive clinical trials were conducted in the 1980s to assess the effect of prophylactic and therapeutic supplementation of vitamin E. Prophylactic oral administration of free vitamin E to babies at high risk for ROP had no effect on incidence, but reduced severity of ROP (73). Three other controlled trials using intramuscular, oral, or variable intravenous doses of vitamin E demonstrated a reduction in incidence, but none of the results were statistically significant (74–76). Unfortunately, despite an abundance of data on the use of vitamin E in the treatment of ROP, there remains no conclusive case for its efficacy. Brion et al. (77
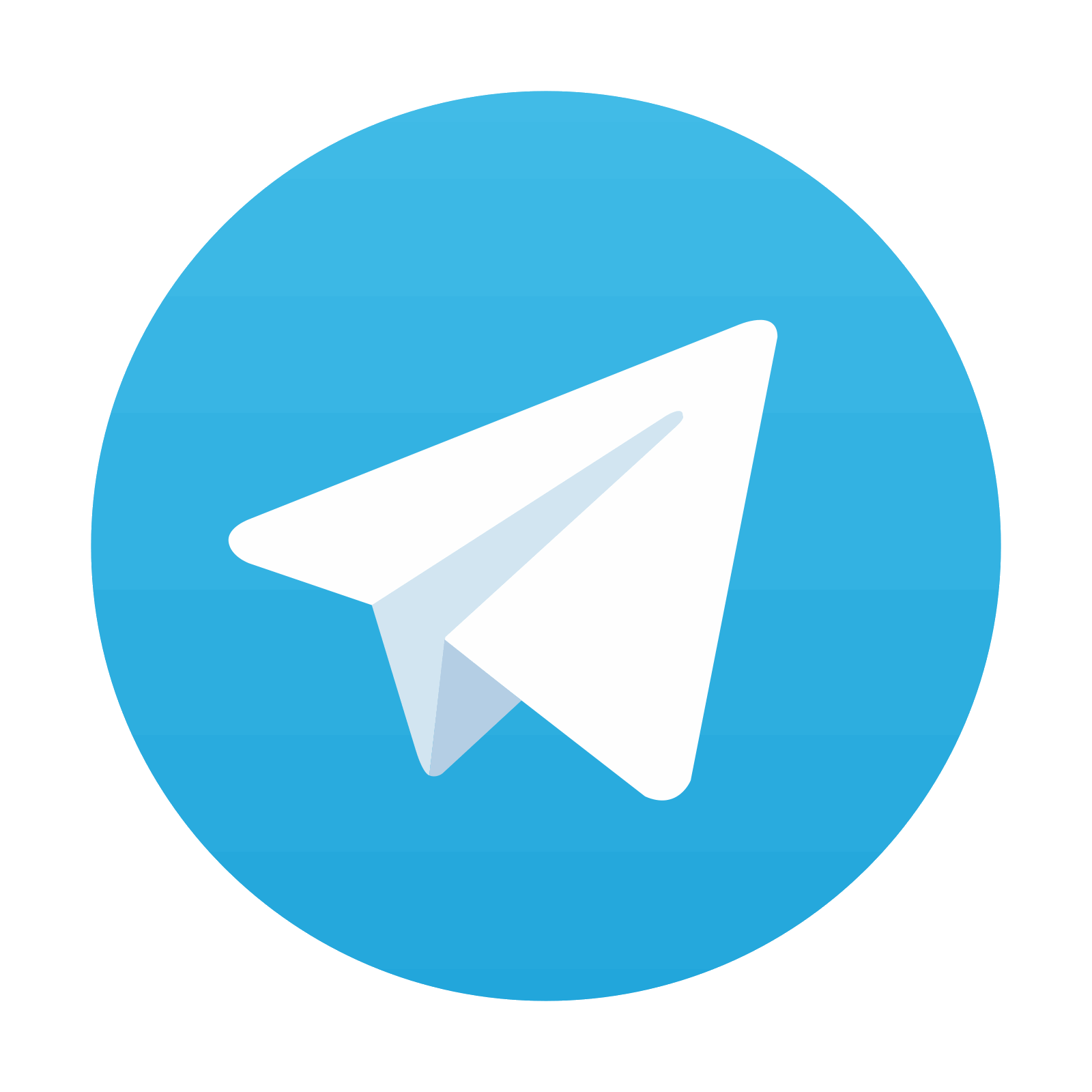
Stay updated, free articles. Join our Telegram channel
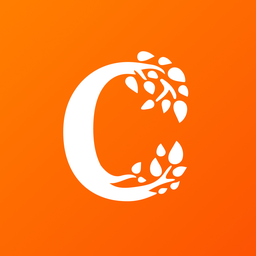
Full access? Get Clinical Tree
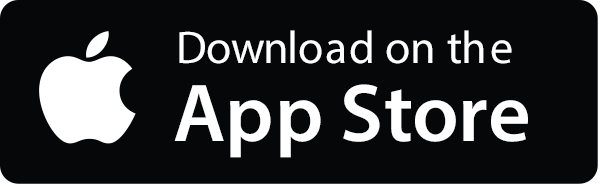
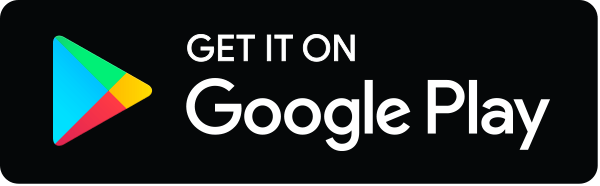