Eberhart Zrenner MD Modern structure-based drug design results increasingly in compounds that act very specifically, e.g. as modulators of the function of channels or enzymes that occur not only in the targeted tissue but also in the eye. Increasingly, therefore, ophthalmological symptoms occur in phases I and II of clinical studies without any ophthalmic signs having been reported in preclinical studies. A well-known case is sildenafil (Viagra®), a selective inhibitor of cGMP-specific phosphodiesterase (PDE) 5 in the corpus cavernosum that, with lower selectivity, also affects the retinal PDE6 and elicits luminous phenomena such as blue-tinged objects and phosphenes, and thus reflects an unexpected pharmacological side effect (for a survey see Laties and Zrenner 2002). In such cases, usually long-term, multicenter studies are required to exclude irreversible effects such as retinal degeneration and permanent loss of certain visual functions. As more and more drugs are targeted at specific molecular actions, e.g. specific protein structures or receptor subfamilies with particular ligand abilities that may occur in the eye as well, specifically targeted tests and study designs have to be used to differentiate between effects merely reflecting harmless pharmacological actions at an undesired site from those that lead to irreversible, toxic damage. Moreover, it is still unclear which battery of ophthalmic function tests assesses the safety of specific drug actions best and how one sets the threshold for potential clinical concern and the proper endpoint for safety. In addition, given the complexity and multitude of stimulation and recording conditions of tests such as electroretinography, electrooculography and visually evoked cortical potentials (VEPs), it is not easy to assure comparability and quality of test results in multicenter trials, not to mention the large variations in procedures, devices and methods of evaluation. This chapter therefore outlines some principles that may help to select proper tests on the basis of their specificity for the function of certain retinal cell subgroups. It gives practical advice for selecting those tests that will enable the investigators to assess the specific functions and morphology of the visual system, and it also addresses questions of safety and efficacy, based on hypotheses that stem from preclinical data where the psychophysics of the visual system are not available. Moreover, new developments are pointed out that help to improve the quality of multicenter studies by proper standard operating procedures (SOPs) and stringent case report forms (CRFs), based on the development of international standards and recommendations in ophthalmology (for electrophysiology, e.g. www.iscev.org/standards/) as well as the development of international networks of certified centers (http://www.evicr.net/). Almost half of all neurotoxic chemicals affect some aspect of sensory function (Croft and Sheets 1989), with the visual system being most frequently affected. Grant and Schuman (1993) in their encyclopedic Toxicology of the Eye list approximately 3000 substances that produce unwanted side effects in the visual system. Numerous transmitters and mechanisms are involved in processing information in photoreceptors and in the many connected neurons transmitting visual information to perceptual centers whose function can be affected by neurotropic agents, drugs, food and environmental compounds. Besides the particular role of glutamate and glutamate receptors in the vertebrate retina (see Connaughton 2014) GABA- and Glycin-receptors (Kolb et al 2014), a multitude of transmitters and neuromodulators is found in the various retinal layers, especially in the more than 25 different types of amacrine cells in the inner plexiform layer (see Table 4.1). Compounds can therefore act specifically on the function and electrophysiological characteristics of these target cells. This specificity often allows the correlation of functional alterations in electrophysiological parameters to the action and adverse reactions of neurotropic compounds. Additionally, there are many nonneuronal cells whose function is important for the integrity of information processing, such as pigment epithelial cells, glial cells and vascular structures, that can be affected by drug action as well. Of further general concern are the factors that determine whether a particular chemical can reach a particular ocular site: concentration and duration of exposure, mode of application and interaction with the various ocular structures, as well as integrity of natural barriers. The blood-retinal barrier may be impermeable under normal physiological conditions (Alm 1992). However, in certain areas of the retina, e.g. around the optic disc, the continuous type of capillaries is lacking and hydrophilic molecules may enter the optic nerve head by diffusion from the extravascular space. Table 4.1 The Various Transmitters and Modulators Present in the Retinal Layers (Modified from Vaney 1990 and Kolb et al 2014) This chapter aims to convey a basic understanding of toxic mechanisms in the visual system by pointing out cell-specific functional changes and typical symptoms in toxic side effects. In an individual case, it is strongly recommended that referenced publications such as Grant and Schuman (1993) and Fraunfelder (2000) are consulted. These have references on the primary literature concerning the various substances. Additionally, there are very interesting general chapters and books that concern ocular toxicology and may be of help (e.g. Chiou 1992; Potts 1996; Ballantyne 1999; Fox and Boyes 2001; Bartlett and Jaanus 2007) also in case of assessing ocular toxicology in laboratory animals (Weir and Collins, 2013) or in case of pesticide exposure (Jaga and Dharmani, 2006). The retinal pigment epithelium (RPE) has four major functions: phagocytosis, vitamin A transport and storage, potassium metabolism and protection from light damage (see Fig. 4.1). RPE functions can be altered, e.g. by inhibitors of phagocytosis, modulation of potassium metabolism, metabolic alterations in the visual cycle and the action of melanin-binding substances. Additionally, melanin is found in several different locations, such as the pigmented cells of the iris, the ciliary body and the uveal tract. Melanin has a high binding affinity for polycyclic aromatic carbons, calcium and toxic heavy metals such as aluminum, iron, lead and mercury (Meier-Ruge 1972; Potts and Au 1976; Ulshafer et al 1990; Eichenbaum and Zheng 2000). This may result in excessive accumulation and slow release of numerous drugs and chemicals that bind to melanin granula, such as phenothiazines, glycosides and chloroquine (Bernstein 1967, Alkemade 1968). The outer retina is supplied by the choriocapillaries; capillaries have loose epithelial junctions and multiple fenestrae and are highly permeable to large proteins (Fig. 4.2a). During systemic exposure to chemicals and drugs by inhalation, transdermally or parenterally, certain compounds can be distributed to all parts of the eye via the bloodstream (Fig. 4.2b). For example, chloroquine phosphate binds strongly to the RPE with a half-life of five years, 80 times more strongly than to the liver. This drug not only is used for malarial prophylaxis, but also plays an important role in the treatment of rheumatoid diseases (up to 4 mg/kg, or for hydroxychloroquine 6 mg/kg, of body weight per day). At these maximal rates, a critical cumulative dose can be reached within 3–6 months (Mavrikakis et al 2003). Signs of chloroquine phosphate retinopathy (typically following a cumulative dose of 100–300 g) are: • loss of blue/yellow color discrimination • reduced light-evoked amplitude rise in the electrooculogram (EOG) Patients with low body weight and/or poor renal function are particularly susceptible (Ochsendorf et al 1993); therefore, care should be taken to avoid overdosage with this melanin-binding drug. In general, if a drug product is found to bind to melanin, it would be important to know whether the nonclinical studies demonstrated abnormalities in electroretinograms (see page 15 in Part 3). Cytostatic agents that disrupt protein metabolism can have an effect similar to the retinal toxicity of the antimalarial drugs. These include vincristine, vinblastine, sparsomycin, triazyquone acting through inhibition of protein synthesis (see Zrenner and Hart 2007) and some neurotoxic antibiotics, such as streptomycin and its associated derivatives, as well as phenothiazines and indomethacin (Palimeris et al 1972). The retinal pigment epithelial cell forms a syncytium with tight junctions that is extremely important for ionic homeostasis of the retina, given the various pumps and channels of the basal as well as of the apical membrane (Fig. 4.2b). Thereby fluid accumulation can occur as toxic side effect, causing edema, especially in the macular region, as can be tested in experimental models as well (Maminishkis and Miller 2010). Such changes are another source of drug-induced functional alterations by changes in standing potential across Bruch’s membrane accessible by electrooculography (see section ‘Functional alterations in photoreptors’, below). The pigment epithelial cell is intricately connected with the photoreceptor function through the visual cycle (Fig. 4.3). This includes several transport proteins between photoreceptors and pigment epithelial cells, as well as enzymes that are important for the renewal of rhodopsin (Strauss 2014). Compounds that affect the transport or metabolism of retinol or retinal can result in functional alteration and retinal toxicity, as seen in oral retinoid therapy (Sieving et al 2001, Messias et al 2010). This may influence the dark adaptation of photoreceptors, accessible psychophysically by threshold measurements (Kurtenbach et al 2006), objectively by monitoring the recovery of electrophysiological signals after bleaching (Messias et al 2010) or by measuring contrast vision (Durst et al 2011). Numerous substances can alter visual function by acting on the visual transduction process (lower part of Fig. 4.3). These include, for example, chloramphenicol, as well as glycosides such as digoxin and digitoxin. Most frequently the complaints in such drug actions are hazy or blurred vision, flickering lights, colored spots surrounded by a halo and increased glare sensitivity. Color vision problems have been confirmed (Rietbrock and Alken 1980; Haustein and Schmidt 1988; Duncker and Krastel 1990), probably acting through the inhibition of the retinal sodium/potassium ATPase. ERG analysis typically reveals a depressed critical flicker fusion frequency, reduced rod and cone amplitudes and increased implicit times as well as elevated rod and cone thresholds (Robertson et al 1966; Madreperla et al 1994). PDE inhibitors, such as sildenafil (Viagra®) can also affect the photoreceptor transduction process, acting on the retinal PDE (Schneider and Zrenner 1986). In therapeutic doses, sildenafil can lead to reversible color discrimination problems, blurred vision, glare sensitivity, blue-tinged borders between bright and dark areas and ERG changes at higher doses (Laties and Zrenner 2002). Many drugs can affect the spectrally different cones in the retina in a slightly different manner, and even minor imbalances in the excitation of short-, middle- and long-wavelength sensitive cones can produce color vision disturbances (see Lyle 1974a,b; Zrenner 1982; Zrenner et al 1982; Jägle et al 2007; Zrenner and Hart 2007). Alterations of color perception therefore belong to early signs of drug-induced functional alterations of the visual system. Approximately 110 million rods and 6 million cones converge through a heavy-duty neuronal processor consisting of horizontal cells, several types of bipolar cells and at least 25 types of amacrine cells, the processed signals being forwarded finally onto ganglion cells (see Fig. 4.4). Of special interest is the ON- and OFF-bipolar cell system: ON-bipolar cells that utilize a metabotropic glutamate receptor in order to change their electrical sign are depolarized on light stimulation of the photoreceptors, while OFF-bipolar cells with their ionotropic glutamate receptor are hyperpolarized on illumination similar to that of the photoreceptors (for a survey see Connaughton 2014). A contrast enhancement system is thus established in the retina where ON-bipolar cells actively signal brightness while OFF-bipolar cells actively signal darkness. In the case of function changes in one of the two systems, not only does a loss of contrast vision occur, but also a selective impact of the ON-response in the ERG (b-wave) or of the OFF-response, elicited after switching off a long duration test light. The rod system has only a single bipolar cell system (rod ON-bipolar cell) not directly connected to ganglion cells, but it utilizes a particular glycinergic amacrine cell (AII amacrine cell) as an intermediary to the ganglion cells. Because rod and cone b-waves can be nicely discerned by dark- or light-adapted ERG, drug effects on these systems can be selectively isolated by noninvasive ERG techniques. The outer and inner layers of the retina have numerous neurotransmitters and modulating substances, e.g. glutamate GABA, glycine, acetylcholine, dopamine, serotonin, substance P, vasoactive intestinal peptide, somatostatin, nitric oxide and even angiotensin-converting enzyme (Zrenner et al 1989, Kolb et al 2014). Altering the metabolism, release or uptake of these substances can affect visual function. The following section discusses several more common examples. Antiepileptic drugs act on the GABA metabolism, and carbamazepine (Tegretol®), phenytoin (Phenhydan®) and vigabatrin (Sabril®), for example, quite commonly alter visual function (Dyer 1985; Besch et al 2000, 2002; Harding et al 2002). These drugs can cause color vision disturbances. Vigabatrin can produce irreversible concentric visual field defects with incidences between 0.1% and 30% (Miller et al 1999; Schmitz et al 2002). Vigabatrin raises GABA levels by irreversibly binding to GABA transaminase, thus preventing the metabolism of GABA. The earliest report of retinal electrophysiological changes in humans associated with vigabatrin treatment is that of Bayer et al (1990). All patients with visual field defects revealed altered oscillatory potential waveforms in the ERG, especially patients with marked visual field defects (Besch et al 2002), which were also visible in multifocal ERGs (mfERGs; Ruether et al 1998). Such changes are usually irreversible (Schmidt et al 2002). In many patients a delayed cone single-flash response was found in the Ganzfeld ERG and a reduced Arden ratio in the EOG. Harding et al (2002) developed a special VEP stimulus with a high sensitivity and specificity for identifying visual field defects. Agents that modulate GABA can be expected to alter the GABAergic functions of horizontal cells and thereby can alter contrast vision and presumably functions of light adaptation as well. Drugs such as fluphenazine, haloperidol and sulpiride can affect the dopamine metabolism and thereby modify retinal function (Schneider and Zrenner 1991). In the arterially perfused eye all three dopamine antagonists increased the rod b-wave, while b-wave latency and implicit time showed no drug-induced changes. On the other hand, the D1 antagonist fluphenazine increased the fast transient ON-component while simultaneously strongly decreasing the OFF-component. In contrast, concentrations of the D2 antagonist sulpiride that had a comparable effect on the fast transient ON-component of the optic nerve response (ONR) did not influence the OFF-component. These findings indicate that D1 and D2 receptors play different roles in the transmission of rod signals at the border of the middle and inner retina. As reported in patch clamp investigations by Guenther et al (1994), application of the receptor antagonists haloperidole, spiperone and SCH23390 reduces calcium influx by between 8% and 77%, while no effect of dopamine itself was observed. The study of Dawis and Niemeyer (1986) in arterially perfused cat eyes suggests that dopamine itself has an inhibitory effect on the rod visual pathway because the rod b-wave amplitude is reduced after dopamine application (for a review see Witkovsky and Dearry 1991; Witkovsky 2004). The action of these drugs may be based on a particular cell population of dopaminergic neurons, the density of which ranges from 10 to 80 cells/mm2. They surround other amacrine pericaria, probably amacrine cells of the AII type that transmit rod pathway information onto cone bipolar cells. Approximately 20 different transmitter substances and modulators are used by the various types of amacrine cells, as shown in Table 4.1. Consequently, numerous drugs can affect the function of amacrine cells, and individual wavelets of the oscillatory potentials that have their origins in amacrine cells can be affected differently. A good example of this is angiotensinergic amacrine cells (Datum and Zrenner 1991; Jurklies et al 1995; Kohler et al 1997; Wheeler-Schilling et al 2001). Angiotensin II antagonists can considerably alter retinal function, as shown in ERGs and ONRs of the arterially perfused cat eye (Dahlheim et al 1988; Zrenner et al 1989) and by electroretinography in cats (Jacobi et al 1994). While the parvocellular system is mainly responsible for coding of fine spatial resolution and color vision, the magnocellular system codes primarily movement and contrast. Drugs that affect ganglion cell function therefore can show different effects on the parvo- and magnocellular systems, e.g. ethambutol. Ethambutol is widely used for the treatment of tuberculosis. It causes: • color vision disturbances as early symptoms • visual field defects, especially central scotomas with loss of visual acuity These alterations can occur within a few weeks after starting the treatment. Also, ethambutol may act at different sites. While initially horizontal cell function and thereby color discrimination are altered as seen in VEPs (Zrenner and Krüger 1981), as well as in fish retina single-cell recordings (van Dijk and Spekreijse 1983), advanced forms may lead to loss of ganglion cells and degeneration of the optic nerve. After cessation of drug intake, color discrimination improves, as can easily be shown by anomaloscopy (Nasemann et al 1989). Other substances that typically modify ganglion cell functions are methanol and ethanol, chloramphenicol, quinine, thallium and ergotamine derivatives (for references see Zrenner and Hart 2007). Several compounds particularly affect axonal fibers of ganglion cells. For example, exposure to acrylamide produces particular damage to the axons of the parvocellular system, while it spares axons of the magnocellular system, leading to specific visual deficits, such as an increase in the threshold for visual acuity and flicker fusion, as well as prolonged latency of pattern-VEPs. The changes induced by carbon disulfide (CS2) on the visual function are central scotoma, depressed visual sensitivity in the periphery, optic atrophy, pupillary disturbances, disorders of color perception and blurred vision (for a survey see Beauchamp et al 1983). Vasculopathies including the retina were reported as well, but histology in animals points primarily to an optic neuropathy (Eskin et al 1988), and alterations in the VEP are expected in such conditions. Kaiser-Kupfer et al (1981) reported widespread axonal degeneration induced by tamoxifen in the macular and perimacular area, accompanied by retinopathy. Clinical symptoms include a permanent decrease in visual acuity and abnormal visual fields (Ah-Song and Sasco 1997). Following cessation of low-dose tamoxifen therapy, most of the keratopathy accompanying tamoxifen intake and some of the retinal alterations were seen to be reversible (Noureddin et al 1999). Other more common drugs that can produce alterations of optic nerve function with concomitant VEP changes are ethambutol, isoniazid, and isonicotinic acid hydrazide, as well as streptomycin and chloramphenicol. The retina has several types of glial cells: Müller cells, oligodendrocytes and astrocytes. Müller cells are important for glutamate metabolism (in the uptake of the transmitter released by photoreceptors), as well as for the storage of calcium ions. Damage to glial cells, e.g. by the ammonia toxicity of alcohol syndrome (caused by severe toxic hepatic damage), produces marked changes in the ERG (Eckstein et al 1997). This arises because of damage to Müller cells, because the b-wave of the ERG is strongly modulated by the function of the retina’s Müller cells. As has been outlined for retinal disorders, toxic and pharmacologic disturbances of visual function can also show up in the central nervous system. In addition to neurotropic effects, some drugs can cause an elevation of intracranial pressure, resulting in papillary edema, which is observed, for example, after the treatment with ergotamines and may produce prolonged latency in the pattern-VEP (Heider et al 1986). Because many cortical areas are involved in processing visual information, alteration of vision can also be caused by direct drug action on neurons in higher cortical areas and produce visual hallucinations and neurological deficits. Noninvasive tests allow the recording of bioelectric potentials that arise during the neural processing of visual information by the various elements of the afferent visual pathways. Changes in the various signals allow conclusions about the locations and kinds of functional disturbances in the afferent neurons of the visual system. Electrophysiology, including the electrooculogram, the Ganzfeld electroretinogram, pattern- and multifocal ERG, as well as visually evoked cortical potentials have been standardized by the International Society for Clinical Electrophysiology of Vision (ISCEV, http://www.iscev.org/standards/index.html). This site also provides further guidance for the proper application of electrophysiological tests. To make valid use of electrophysiology, it is necessary to know the anatomic origins of the various potentials, the appropriate stimuli to evoke them and the methods for recording and measuring their responses. Table 4.2 shows a survey (modified after Apfelstedt and Zrenner 2007) arranged according to the various anatomic structures of the visual pathway. Nearly every part of the visual pathway can be studied with at least one electrophysiological method. Table 4.2 Origin of the Various Components of the Electrophysiological Recordings in Relation to Morphological Sites of the Retina (Modified after Apfelstedt and Zrenner 2007) The EOG measures a physiological electrical potential difference between the cornea and the posterior pole of the globe, with the cornea by convention being the positive pole. This so-called ocular resting potential is a physiological, transepithelial electrical potential that is present across the RPE, as shown in Fig. 4.2b. Under steady conditions of illumination, the resting potential maintains a constant value called the baseline potential, but it will vary with changes in illumination. An increase in this potential is a response of the RPE to light-induced shifts in ion concentrations in the extracellular fluid of the photoreceptor, induced by the activation of the phototransduction process in response to illumination of their outer segments. The light-dependent changes in the ocular resting potential recorded by the EOG require an intact function of the RPE and the photoreceptors. The EOG is recorded by means of a pair of skin electrodes fixed close to the canthi of the eyelids. The patient is asked to continuously alternate his/her gaze between fixation lights at 1 second intervals for 10 seconds every minute. The fixation lights are separated by a visual angle of 40° from each other and located in a diffusely illuminated Ganzfeld sphere. The gaze-induced change in the eye bulb’s angle alters the relative potential difference between the nasal and temporal electrodes. The gaze-induced potential difference is directly related to the size of the ocular resting potential. The gaze-induced modulation of the potential is measured for about 30 minutes. The EOG procedure is described in the ISCEV Standard by Marmor et al 2011. Dark adaptation of the light-adapted eye results in a decrease in the ocular resting potential, which reaches a minimum value after about 10 minutes, the so-called dark trough (Arden et al 1962). During subsequent steady illumination of the eye, a continuous rise in the resting potential is evoked, which reaches the so-called “light peak” 8–10 minutes after light onset (lower line in the right part of Fig. 4.5). The essential measure of the EOG is the ratio between light peak amplitude and dark trough amplitude just before light onset, the Arden ratio, which typically amounts to about 1.5–2.5 (right part of Fig. 4.5). Additionally it is possible to evoke a fast oscillation by setting a light on and off every 75 seconds. This potential is shown on the left of Fig. 4.5 and magnified in the inset figure. It stems from the RPE basal membrane. Widespread damage to the pigment epithelial/photoreceptor complex results in a diminished light/dark ratio up to a complete loss of the light-induced potential increase. Due to the origin of the standing potential, the EOG is the test of choice in cases where the primary functional alteration is expected in RPE cells, e.g. in chloroquine retinopathy (Johnson and Vine 1987) or phenothiazine retinopathy (Henkes 1967). Because the light-induced increase in the standing potential is triggered by photoreceptor excitation, an intact function of the neuroretina is required to identify a pigment epitheliopathy at an early stage. Electroretinographical recordings must therefore accompany the EOG tests as well as careful ophthalmoscopy of the pigment epithelial layer for biomicroscopic alterations. Biomicroscopy is supported by autofluorescence measurements and fluorescence angiography, methods which allow us to assess the state of the RPE layer more accurately. Illumination of the entire retina by a short flash causes all retinal neurons to respond with a change in their electrical potential, triggered by the phototransduction process that finally modulates the glutamate release in the photoreceptor synapses. The sum of light-evoked changes in the membrane potential of retinal cells can be recorded by corneal electrodes, such as contact lenses, gold foils or fiber electrodes, resulting in the ERG. The sketch in Fig. 4.6 points out the light-induced increase in extracellular potassium (K+) in the outer and inner plexiform layers that is picked up by millions of Müller cells. Each Müller cell directs the potassium current toward the vitreous humor, forming a current loop mainly induced by the activity of the depolarizing bipolar cells (Fig. 4.6). While the first event in ERG response to a strong flash is a negative response (the a-wave, shown at the top of Fig. 4.7) that reflects the photoreceptor outer segments at least in the first 10–12 milliseconds, the subsequent positive response (b-wave) is mainly created by the depolarizing bipolar cells, whose excitation is reflected in the Müller cell currents, picked up as b-waves by corneal electrodes. The various types of amacrine cells contribute to the oscillatory potentials that are visible as little wavelets on the ascending limb of the b-wave and can be made visible by filtering out the slower a- and b-waves electronically or digitally.
The role of electrophysiology and psychophysics in ocular toxicology
Introduction
The Visual System
Neuroactive Substance
Cell Type
Glutamate
Photoreceptors (cones and rods), bipolar cells, ganglion cells
Gamma aminobutyric acid (GABA)
Horizontal cells, amacrine cells
Glycine
Amacrine cells, bipolar cells, ganglion cells
Taurine
Photoreceptors, amacrine cells, bipolar cells
Dopamine
Amacrine cells (including interplexiform cells)
Melatonin
Photoreceptors
Serotonin
Amacrine cells, bipolar cells (in nonmammalian vertebrates)
Acetylcholine
Amacrine cells (in the INL and displaced in the GCL)
Substance P
Amacrine cells, ganglion cells
Angiotensin II
Amacrine cells
Nitric oxide
Amacrine cells
Vasoactive intestinal polypeptide (VIP)
Amacrine cells
Somatostatin
Amacrine cells, ganglion cells
ATP
Amacrine cells, ganglion cells
Adenosine
Amacrine cells, ganglion cells
Brain-derived neurotrophic factor (BDNF)
Amacrine cells, ganglion cells
Kynurenic acid
Amacrine cells
The retinal pigment epithelium
Functional alterations in photoreceptors
Functional alterations in cells of the inner retina (from outer plexiform to inner plexiform layer)
GABA and antiseizure medications
Dopamine
Other retinal transmitters and modulators
Functional changes in retinal ganglion cells
The optic nerve
Carbon disulfide
Tamoxifen
Other toxic optic neuropathies
Damage to glial cells
CNS damage
The Role of Noninvasive Electrophysiology in Drug Testing
Relationship between anatomy and function
Anatomic Structure
Electrophysiological Potential
Clinical Test
Retinal pigment epithelium and photoreceptor outer segments
Ocular resting potential
Electrooculogram (EOG)
Rod outer segments
Rod a-wave
Scotopic flash ERG
Cone outer segments
Cone a-wave
Photopic flash ERG
Rod ON-bipolar cells
Rod b-wave
Scotopic flash ERG
Cone ON-bipolar cells
Cone b-wave
Photopic flash ERG
Rod OFF-bipolar cells
Rod off-effect
Scotopic long duration flash ERG
Cone OFF-bipolar cells
Cone off-effect
Photopic long duration flash ERG
Müller glial cells
Rod and cone b-wave
Scotopic and photopic flash ERG
Inner plexiform layer and especially amacrine cells
Oscillatory potentials
Scotopic flash ERG, with special filtering
Ganglion cell layer
N 95 potential
Pattern ERG
Posterior pole, cones, bipolar cells and amacrine cells
Local retinal responses
Multifocal ERG
Retinocortical conduction time
Latency/implicit time
Visually evoked potentials (VEP): pattern-VEP
Primary visual cortex and the cone dominated portion of the afferent pathway (central visual field)
P100 amplitude, P2 amplitude
Pattern-VEP, flash VEP, multifocal VEP
Electrooculography
Ganzfeld electroretinography
4 The role of electrophysiology and psychophysics in ocular toxicology
Part 4
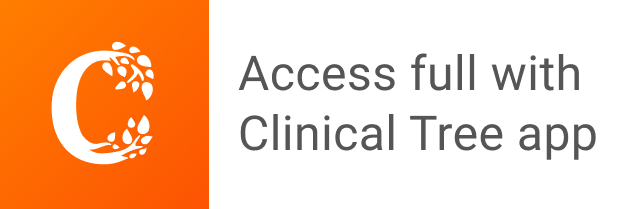