Summary
Current surgical approaches overemphasize repositioning the tendinous insertions of the extraocular muscles (EOMs), neglecting key concepts about EOM anatomy that provide the foundation for both innovations in surgical technique and enduring improvement in eye alignment and movement. For example, the EOM paths through the orbit from apex to globe are highly stereotypical among normal subjects; a 2- to 4-mm deviation in path is sufficient to trigger abnormal function. The muscle paths are constrained by fibromuscular bands of connective tissue, pulley sleeves that can be affected by many diseases as well as an overall deterioration in stability with age. Changes in pulley sleeve strength and positioning destabilize normal muscle function and create imbalances in muscle forces that lead to disorders in eye alignment and movement, disorders that can be treated by strengthening and repositioning the pulley sleeves rather than repositioning the tendinous insertions. Likewise, most EOMs contain bifid, segregated neuromuscular innervation, effectively acting as two independent muscle compartments within a single muscle belly. This segregated innervation is especially important to consider when evaluating superior oblique function because an asymmetrical compartmental palsy of the trochlear nerve could preferentially affect either torsional or vertical eye alignment. Finally, each EOM has two functional layers. The outer, orbital layer inserts onto the connective tissue pulley sleeve rather than the globe, while the inner, global layer inserts upon the globe. Trauma to the orbital connective tissue that spares the muscle can still impede function by displacing pulley locations and restricting pulley movements.
4 Surgical Anatomy of the Extraocular Muscles
4.1 Anatomy Is the Foundation for Function
Normal extraocular muscle (EOM) function depends on intact innervation, properly constrained and positioned muscle paths, and anatomically accurate and firm tendinous attachments onto the globe. Normal motility on clinical exam, however, does not depend entirely on normal anatomy.
4.1.1 The Role of Fusion
Fusional mechanisms can compensate for many defects in EOM function, especially if the defects are small or slowly increase over time. A classic example is the large vertical fusional ability, virtually nonexistent in normal people, that can be measured in patients with congenital superior oblique (SO) palsies. 1 Fusion to maintain normal motility is critically important in all people, however, because the globe and orbit continually change over the natural human life span. From infancy through adolescence into adulthood, growth and elongation of the globe changes the relative positions of the EOM insertions, while increasing orbital depth and volume also change the lengths and paths of the EOMs. 1 In the elderly, senescence brings diminished connective tissue strength and elasticity with corresponding sag of both globe and pulley sleeves, introducing yet another destabilizing element that can affect motility. 2 , 3 For the majority of individuals, fusional mechanisms compensate for the shifting anatomy, resulting in normal binocular vision throughout life.
4.1.2 The Role of Orbital Anatomy
Fusion, in turn, is aided by two key anatomical constructs: 1) fibromuscular connective tissue bands form pulley sleeves that guide the rectus EOMs through the orbit, minimizing sideslip during gaze changes 4 ; and 2) a portion of each rectus EOM, the orbital layer, inserts onto the pulley sleeve instead of the globe, dynamically shifting the pulley position posteriorly during contraction and anteriorly during relaxation 5 , 6 (Fig. 4‑1). These two constructs dramatically decrease the neurologic complexity for controlling eye movements, in essence implementing much of the complex rotational mathematics required for coordinating three-axis eye movements mechanically in the orbit without requiring higher-level input. 5 , 6 , 7 , 8

Abnormalities in EOM paths or in the active anteroposterior movement of the pulley sleeves disrupt normal orbital biomechanics and dramatically increase the difficulty of controlling eye movements. Whether the disruption creates a problem with binocular vision, in turn, depends on two additional factors: the severity of the abnormality and the fusional ability of the affected person. Multiple, severe pulley positional abnormalities (e.g., craniosynostosis syndromes) create obvious impairment of normal eye movement, 1 but milder displacements of single pulleys can be completely compensated for until an event like a concussive head injury impairs fusion and unmasks the underlying instability. Likewise, an entrapped inferior rectus (IR) pulley after an orbital blowout fracture creates an immediate restriction and diplopia, 1 but lesser degrees of trauma could result in a flap tear within the muscle with scarring that can evolve into strabismus over months or years. 9 Finally, defects in fusional ability from childhood strabismus, anisometropia, or decreasing vision caused by cataract formation or age-related macular degeneration could relatively suddenly change a long-standing, controlled anatomical defect into a clinically obvious strabismus.
In summary, the anatomy of the EOMs is uniquely and elegantly designed to simplify neurologic control of binocular eye movements. Disruption of that anatomy erodes the biomechanical foundation for coordinated eye movements, potentially overwhelming fusion to create clinically important strabismus.
4.2 The Globe Center Is the Key Landmark
“Eye movement” is a misnomer—the eye translates less than a millimeter, on average, toward a contracting EOM during changes of gaze. 10 Instead, the eye rotates to a position of gaze that balances the totality of all the EOM forces acting upon it. Each EOM potentially contributes horizontal (abducting and adducting), vertical (supraducting and infraducting), and torsional (incycloducting and excycloducting) forces onto the globe depending on relative position of each muscle’s direction of force (its force vector) with respect to the globe’s rotational axis, which on average is approximately 1 mm medial and 0.5 mm anterior to the actual center of the globe.
The pulley sleeves dramatically simplify the effect of EOM forces on the globe by centering the force vectors near the globe’s rotational axis in either the horizontal or vertical plane. When properly centered, the horizontal rectus muscles, in particular, exert minimal vertical or torsional forces onto the globe during contraction (Fig. 4‑2a). Eccentric pulley positions, on the other hand, add confounding vertical and torsional forces onto the globe in direct proportion to the magnitude of the displacement from globe center (Fig. 4‑2b). 11 A 2- to 4-mm displacement appears to be the threshold for a clinically important effect, diverting 10 to 15% of the contractile force away from the muscle’s primary action into secondary rotational axes.

The pulley suspensions are circumferential and couple the horizontal to the vertical rectus muscles both medially and laterally. The medial rectus (MR) is coupled to both the SO and the superior rectus (SR), as well as to the trochlea and medial orbital wall. The lateral rectus (LR) is mainly suspended from the SR, a connection labeled the LR–SR band. Likewise, the MR connections to the SR are labeled the MR–SR band. The IR is coupled to the inferior oblique (IO), as well as the MR (MR–IR band). Although there is a layer of tissue between the LR and IR, it surrounds the IO and is not well defined and therefore is not named. There are differences in density between these couplings, with the MR–SR band the densest and the LR–SR band the most defined on imaging.
4.2.1 Detecting Pulley Position Abnormalities
Currently, it is not possible to detect anatomical abnormalities in EOM pulley positions using only the clinical exam. Facial asymmetry or measurable displacements of externally visible landmarks like the lateral canthus do not reliably correlate with displacements of the pulleys, although the presence of such abnormalities should alert the clinician to the possibility of a pulley positional abnormality. 12 Noninvasive imaging, on the other hand, can readily detect clinically significant pulley displacements 11 , 13 , 14 and should be considered part of the work-up for incomitant or unusual presentations of strabismus.
4.2.1.1 Imaging Technique
Magnetic resonance imaging (MRI) is the current preferred choice for orbital imaging due to the impressive soft tissue resolution without ionizing radiation. A major limitation, however, is the extended time required to obtain high-resolution images. Small shifts in head or eye position during image acquisition can degrade the images with motion artifacts. The highest-quality images are obtained using direct coronal image planes with the patient’s head stabilized and gaze controlled by fixation targets, preferably in central gaze, using T2-weighted fast spin echo techniques. 15 Additional enhancements in image quality can be obtained by centering a small field of view on each orbit separately and using surface coils to increase the signal-to-noise ratio 15 (Fig. 4‑3).

4.2.1.2 Image Analysis
Once the images are acquired, extraorbital landmarks like the interhemispheric sulcus should be used to place the images into the correct anatomical orientation. 10 Then, image planes that include well-defined cross sections of each of the rectus EOMs and also the globe should be analyzed (Fig. 4‑3). Horizontal and vertical lines bisecting the globe center should also transect about one-third of each rectus EOM (Fig. 4‑3). If those lines do not touch any part of a muscle, it may be displaced more than 2 standard deviations from its normal position and deliver clinically important secondary forces onto the globe during contraction. 14
4.2.2 Determining the Biomechanical Effects of Pulley Position Abnormalities
The biomechanical effects of pulley abnormalities can be divided into two broad groups: (1) central gaze abnormalities caused by an imbalance in muscle forces across the globe center and (2) incomitant eye movements that occur when EOM contraction toward a displaced pulley rotates the eye out of its intended path. If the entire orbit is rotated (e.g., in craniosynostosis syndromes), agonist–antagonist rectus EOMs can be symmetrically displaced from their normal positions with respect to globe center with no resulting central gaze imbalance in muscular forces. During attempted horizontal and vertical gaze changes, however, the abnormal pulley positions cause secondary deviations in globe rotation that can mimic oblique muscle dysfunction. 14 Similarly, A or V patterns of incomitant strabismus can also be caused by congenital or acquired displacements of EOM pulleys without invoking oblique muscle dysfunction. 14 On the other hand, when only a single muscle is displaced, as can occur in sagging eye syndrome for the LR muscle, 2 , 3 the loss of 10 to 15% of central gaze LR force may be sufficient to cause a distance esotropia without introducing a clinically important deficit to abduction.
4.3 There Are 11 Extraocular Muscle Compartments Acting on Each Eye
Each of the EOMs except the SR and levator palpebrae superioris contains two segregated compartments of neuromuscular innervation 16 , 17 , 18 (Fig. 4‑4). Because the parallel muscle fibers are relatively mechanically independent 19 , 20 and most of the EOM compartments have demonstrated independent contraction and relaxation in vivo, 21 , 22 , 23 the globe effectively has 11 different EOM compartments acting upon it.

4.3.1 Superior Oblique Muscle
The SO muscle has the most biomechanically complex anatomy. Clinically, the anterior and posterior halves of its tendinous insertion have long been considered to have different functions: its anterior half inserts near the globe equator and delivers more incycloducting force onto the globe, while its posterior half inserts posterior to the equator and delivers more infraducting force onto the globe (Fig. 4‑5). 17 The discovery of segregated compartmental innervation, however, making it possible to neurologically isolate those two functions and control them independently, is more recent. 17

The SO is actually a strap EOM, just like the other rectus muscles, rolled into cylindrical form to pass through the trochlea. 17 The trochlear nerve bifurcates prior to entering the muscle belly, dividing the innervation into medial and lateral neuromuscular compartments. 17 The medial nerve branch selectively innervates muscle fibers that insert near the equator, controlling incycloduction, 17 while the lateral nerve branch selectively innervates muscle fibers that insert posterior to the equator, controlling infraduction 17 (Fig. 4‑5). The separation into medial and lateral compartments is not anatomically vertical, with the bisector defining the two compartments offset from the vertical long axis of the muscle belly by approximately 30 degrees (Fig. 4‑4).
The two independent neuromuscular compartments within the SO help explain the variety of clinical presentations that can result from a trochlear nerve palsy. Rather than behaving as a single, monolithic muscle, selective atrophy of one compartment might create a greater vertical imbalance than torsional imbalance, or vice versa. 24 , 25 Orbital imaging can aid in the diagnosis, with the type of SOatrophy observed on coronal imaging correlating with complete versus compartmental trochlear nerve paresis. 24 , 25 An atrophic, round muscle belly correlates with a complete palsy, while an atrophic, elongated muscle belly correlates with a compartmental palsy (Fig. 4‑6). 24 , 25

4.3.2 Medial Rectus and Lateral Rectus Muscles
The MR and LR have vertically segregated compartments (Fig. 4‑4). Because their broad tendinous insertions allow for a significant offset of applied force above and below the globe center, each compartment has the potential for clinically important vertical and torsional actions even if the pulley sleeves are in the normal positions, but only if the compartments act independently. Such behavior has already been demonstrated for the MR superior compartment, which is more contractile during conjugate adduction than converged adduction, 26 and for the LR inferior compartment, which demonstrates more contraction than the superior compartment during ocular counter-rolling. 21 In addition, a subset of sixth nerve palsies, LR superior compartment palsies, has recently been identified where a partial palsy of the sixth nerve spares the LR inferior compartment. 27 Patients with this diagnosis have a smaller central gaze esotropia and less reduction in abducting force than those with a complete LR palsy; most importantly, these patients often exhibit small central gaze hypotropias from the unbalanced LR force without the presence of a concurrent vertical muscle palsy. 27
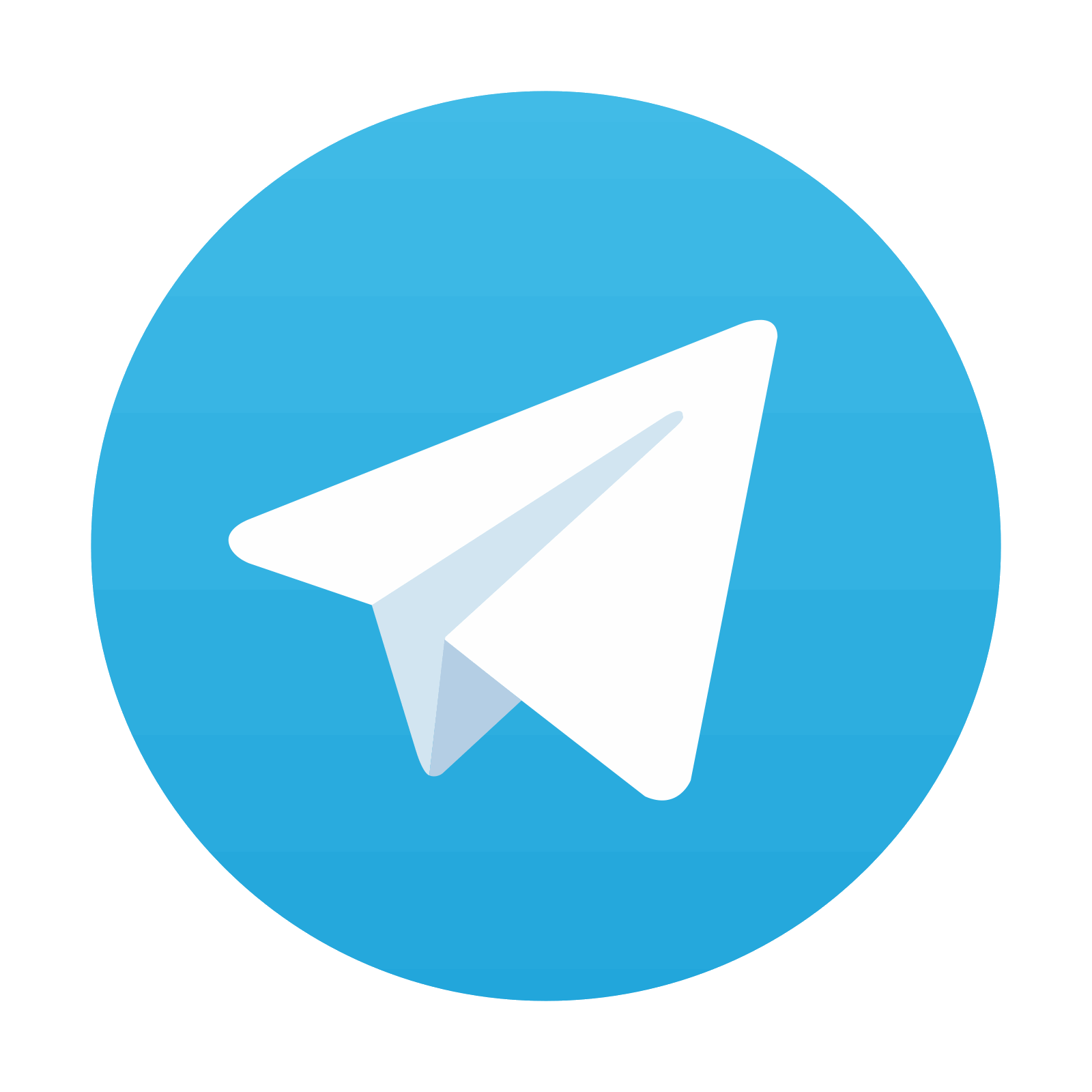
Stay updated, free articles. Join our Telegram channel
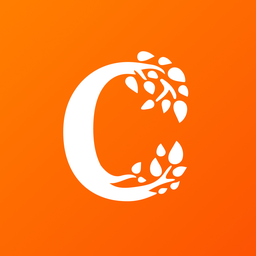
Full access? Get Clinical Tree
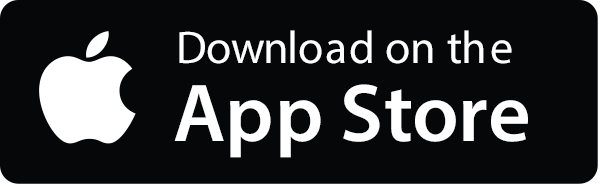
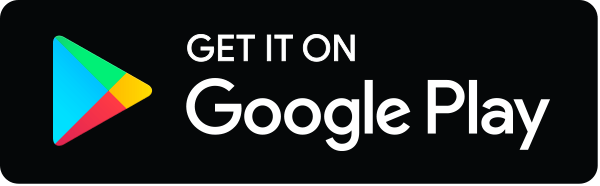
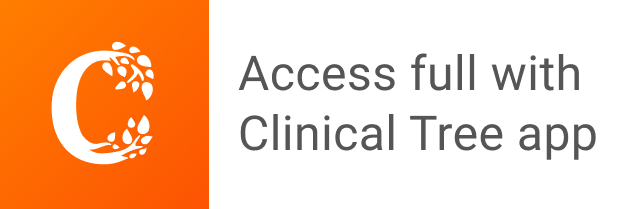