Beneficial, Innately Complicated
Matthew Neu • Ashay Bhatwadekar • Reinhold Medina • Alan Stitt • Maria Grant
INTRODUCTION
The notion of using endothelial progenitors to repair vasodegenerative vasculature in diabetic retinopathy is an interesting alternative to current treatment for retinopathy, the leading cause of visual impairment in the Western world.1 This complication poses serious therapeutic problems and, even with current management regimens, continues to significantly reduce the quality of life for millions of people. Diabetic retinopathy is thought to be a result of hyperglycemia-induced retinal microvascular dysfunction, although there is growing evidence that a significant retinal neuroglial dysfunction also occurs during diabetes, perhaps in unison with vascular pathophysiology.2 The vasodegenerative phase of diabetic retinopathy consists of significant autoregulatory dysfunction, blood flow abnormalities, premature death of capillary components, and eventually widespread nonperfusion of the inner retina.3 Proliferative diabetic retinopathy ensues when the ischemic insult is sufficient to trigger retinal neovascularization, which is superimposed upon “background” retinopathy.
Currently available treatments for diabetic retinopathy such as panretinal laser photocoagulation, vitreoretinal surgery, and recently introduced vascular endothelial growth factor (VEGF) inhibitors are mainly focused on end stages of the disease. Importantly, these therapies do not address the primary pathology of retinal neurovascular degeneration during diabetes that precedes the sight-threatening neovascularization and diabetic macular edema. Fresh perspectives on the cellular and molecular mechanisms of diabetic retinopathy could lead to novel and much more effective prevention/reversal strategies. One exciting possible strategy is targeting the early and intermediate stages of vasodegeneration to enhance vessel repair, reverse ischemia, and prevent progression to the late, sight-threatening stages of diabetic retinopathy. For diabetic patients with an occluded retinal microvasculature, measures to preserve surviving vasculature and revascularize defunct capillary beds could extend the lifetime of the neuropile, reduce pathogenic output of vasoactive and neuropathic agents, and ensure retention of serviceable vision.
In the last decade, it has become established that endothelial progenitor cells (EPCs), as a minor subpopulation of the mononuclear cell fraction in peripheral blood, can be recruited to sites requiring vascular repair and contribute to the viability of the vasculature.4 These cells are derived from the bone marrow or the vasculature itself and they form the basis for potential therapy based on inducing microvascular repair and/or “therapeutic angiogenesis”(Fig. 27.1). Current clinical trials have shown that intramyocardial injection/intracoronary infusion of autologous progenitor cells in patients with intractable angina/acute myocardial infarction is feasible, safe, and beneficial. The same scenario is also a possibility for treatment of retinal ischemia in diabetic retinopathy, and this review focuses on the latest research on the role of these progenitor populations in this complication. How can this knowledge, largely gained in other vascular beds, be exploited for the benefit of diabetic patients with this vision-threatening complication? The answer may lie in a synthesis of both clinical and basic perspectives now believed to dominate the field and new knowledge gained from advances in stem cell biology.
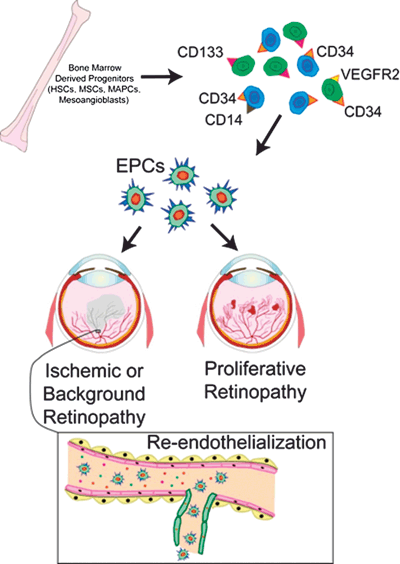
FIGURE 27.1. Role of EPCs in diabetic retinopathy. Bone marrow–derived EPCs in the peripheral circulation express combinations of many surface markers such as CD34, VEGFR2, and CD133. EPCs are home to sites of retinal ischemia and show capacity for repair of injured endothelium in the vasodegenerative stages of diabetic retinopathy. At the later vasoproliferative stages of diabetic retinopathy, EPCs in unison with resident ECs can participate in neovessel formation. Diabetes induces EPC dysfunction, under such circumstances CD14 cells expressing low CD34 can compensate for endothelial repair; however, progressive diabetes converts CD14 cells into an inflammatory, pro-oxidant, eNOS inhibitory, senescent phenotype generating cells that not only repair vasculature poorly but also actually hinder repair and function. HSCs, hematopoietic stem cells; MSCs, mesenchymal stem cells; MAPCs, multipotent adult progenitor cells.
OVERVIEW OF ENDOTHELIAL PROGENITOR POPULATIONS
During the development of the embryo, local mesodermal precursors differentiate into vascular and endothelial cells (ECs) to form a primary vascular plexus, a process referred to as vasculogenesis.5 This process of vasculogenesis was believed to occur only during embryonic development, but not in postnatal life. In the early 1960s, “GORE-TEX” grafts were developed. These artificial grafts were not re-endothelialized by ECs from the neighboring vessel but rather from circulation.6 This gave rise to the idea that circulating cells could form capillaries de novo or serve to re-endothelialize damaged blood vessels. These initial observations were then explained by two groups reporting that human CD34+ cells isolated from circulating peripheral blood, umbilical cord blood, and bone marrow could differentiate into ECs in vitro and in vivo in mouse models.7,8 These studies launched the field that now has advanced to the therapeutic use of EPCs to enhance tissue and vascular repair in patients with ischemic conditions. Furthermore, different studies suggest that incorporation of bone marrow–derived cells occurs in blood vessels. These cells support the maintenance of such vessels9 by healing wounds (Fig. 27.2),10 areas of endothelial denudation,11 induced hindlimb ischemia12 and following experimental myocardial infarction.13 Evidence from patients who have undergone bone marrow or peripheral blood stem cell transplantation shows that some ECs that line blood vessels in the recipient are of donor origin, pointing to the existence of circulating cells in the blood and bone marrow that contribute to endothelial turnover in blood vessels of humans.14
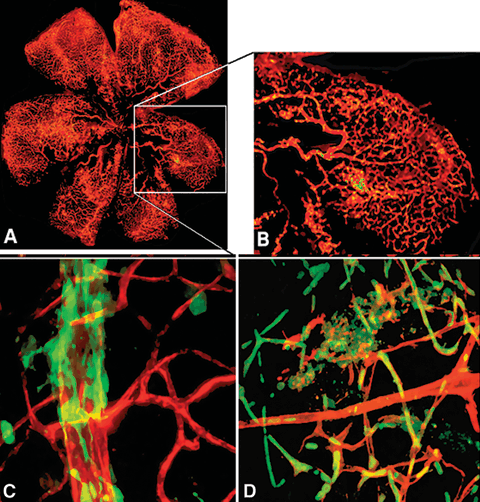
FIGURE 27.2. Fluorescence micrographs showing C57BL/6. gfp chimeric mice that underwent retinal ischemia. A: Typical whole retina from a treated eye. B: Areas with newly formed blood vessels are shown as small regions of green-yellow. C: An area depicting a parent vessel perfused with rhodamine-labeled dextran (red) with extravasations and initial tube formation by gfp+ cells. D: Treated retina showing different stages of new capillary formation by gfp+ cells. (Grant, et al. Nat Med. 2002;8:607–612.)
The use of “EPCs” has extended to a biomarker for vascular health. Clinical studies suggest an inverse correlation between the number of peripheral blood EPCs and the presence of atherosclerosis, an adverse cardiovascular risk score, level of cardiovascular dysfunction, or cardiovascular morbidity and mortality risk.15 Also, the functions of EPCs such as cell adherence, migration, invasion, and vessel formation appear to be reduced in patients with increased cardiovascular risk factors and established cardiovascular disease.16 Therefore, following the numbers of circulating EPCs may help in monitoring the effects of primary and secondary cardiovascular prevention strategies.
In spite of the growing number of reports on EPCs as a potential tool in regenerative medicine or a therapeutic target in oncology, contradicting results have been reported at the preclinical and clinical levels. Most of this confusion is due to varying definitions, identifications, and characterizations of the role of these cells in vascular homeostasis and disease. This chapter discusses these issues in depth and focuses on published studies as well as the authors’ opinions on the potential role of these cells to correct retinal ischemia.
PROGENITOR CELLS IN DIABETES
Both Type 1 and Type 2 diabetic individuals with vascular complications would potentially benefit from cellular therapy with autologous cells. However, bone marrow–derived progenitors from diabetic patients are dysfunctional, producing fewer ECs with reduced proliferative and migratory potential.17 Enhanced oxidative stress in diabetes contributes to progenitor dysfunction.18 Accumulation of reactive oxygen species (ROS) increases cellular and replicative senescence in these progenitors as does increased angiotensin II, oxidized low-density lipoprotein (ox-LDL), and homocysteine. EPCs of diabetic origin show a reduced ability to integrate into EC tubes in vitro compared to EPCs of nondiabetic origin.19–22 Vascularization is depressed when EPCs from diabetic mice are injected into normal mice.23 Recently, it has been shown that activation of the HDL receptor is protective to EPCs by increasing eNOS,24 whereas activation of the ox-LDL receptor downregulates eNOS, supporting a key role for NO in the function of progenitors.25,26
There is a significant depletion of ECs in retinal capillaries during diabetes. The endothelium can correct such loss through increased cell replication (whereby dead cells can be replaced by near neighbors); nevertheless, the concept of the “Hayflick limit” indicates that replicative senescence will occur more rapidly in diabetic retinal capillaries leading to acellular capillary formation. We believe that development of acellular capillaries may be due to a diabetes-mediated dysfunction of EPCs, which manifests as failed attempts at repair of injured capillaries. This leads to the initiation and persistence of ischemia. Research conducted during the past 10 years has focused on understanding the basic mechanism responsible for the diabetes-associated defect in EPC function. Why is this important? Correcting these defects may allow the use of a diabetic patient’s own EPCs for repair of their injured retinal and systemic vasculature as an autologous cell therapy strategy. Specifically in the retina, correction of this dysfunction could treat early and intermediate stages of vasodegeneration to enhance vessel repair, reverse ischemia, and prevent progression to the sight-threatening stages of diabetic retinopathy. However, if circulating cells such as EPCs are given at the “wrong” time, they could exacerbate diabetic retinopathy by contributing to proliferative retinopathy. Moreover, it has been suggested that transplanting some EPC populations into diabetic mice can provoke their differentiation to a proinflammatory and antiangiogenic phenotype and exacerbate ischemia.27 Therefore, transplanting the wrong cell type (perhaps one carrying monocytic markers) into the diabetic retina could enhance differentiation toward an inflammatory cell fate and therefore promote retinopathic progression.
Thus, before EPCs can be used therapeutically in diabetic retinopathy to re-endothelialize acellular capillaries and eliminate retinal ischemia, several key questions must be answered. What triggers this phenotypic change in diabetic cells changing them from reparative cells to cells that are ineffectual at repair? What is the best reparative EPC population? Are some subpopulations more resistant to the injurious effects of diabetes? Should the bone marrow be a target for diabetic retinopathy therapy? What are the “component” cells that contribute to vasculogenesis in the adult? What strategies exist to assess the in vivo function of these diverse cell types?
Specifically in patients with vascular disorders such as diabetes, in vivo function is key. Moreover, confusion in defining functional characteristics and subpopulations of EPCs is due to variable approaches undertaken in research laboratories with regard to the source of the cell, (species and location: bone marrow or peripheral blood) the purification of the cell, the animal model or assay used to test the function of the cell, the method of detection of the cell in vivo and in vitro, and finally the actual interpretation of these studies.
PROGENITOR POPULATIONS DEFINED
There exist two main approaches to define and/or isolate cells with endothelial characteristics and regenerative capacity, as shown in Figure 27.3: culture selection from mononuclear cells (either from bone marrow or peripheral blood) or the use of a set of antibodies to various “identifying” markers of these cells.
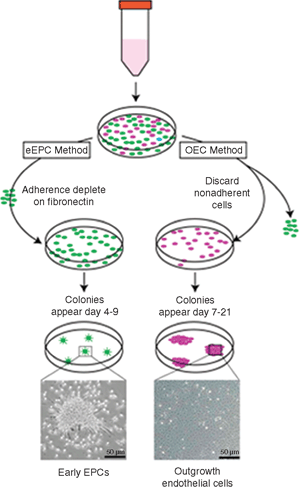
FIGURE 27.3. Isolation and culture of early and late EPCs: Mononuclear cells in presence of selective culture media and matrix (e. g., fibronectin) give rise to eEPCs at 7 to 10 days, whereas OECs are derived later in culture on collagen at 14 to 28 days in endothelial growth conditions. OECs subsequently reach confluence and form tubes on three-dimensional extracellular matrix.
Many groups have characterized endothelial precursors using a panel of immunological and nonimmunological markers in freshly isolated cells, followed by the use of these sorted cells in vitro or in vivo studies. This is the approach commonly used for the isolation of cells (from a patient’s own bone marrow or peripheral blood) for cell therapy. The combination of CD34 and VEGFR-2 as surface markers remains the most common approach for the clinical isolation of these cells, but several other markers have been used to refine the identification of this therapeutic cell type. Some have described CD133 (prominin) as an additional marker,28 particularly to identify the more “immature” EPCs. Considering the uncertainty as to the most reliable method to identify circulating EPCs, most studies employ either the CD34/VEGFR-2 combination or the CD34/CD133 set of markers to quantify circulating EPCs and to correlate their concentration to clinical conditions.29–31
However, turmoil developed in the field when Case et al. reported that isolated human umbilical cord blood or mobilized adult peripheral blood CD34+VEGFR-2+CD133+ cells actually represent an enriched population of CD45+ hematopoietic precursors rather than endothelial progenitors. They cleverly used in vitro hematopoiesis assays to show that CD34+VEGFR-2+CD133+ were hematopoietic precursors and did not contribute to the formation of ECs.32 Others have identified CD34+CD45+CD146+ cells as the true EPCs and thus by using the hematopoietic marker CD45 with the endothelial progenitor markers CD34 and CD146 they believe that the optimal therapeutic cells can be identified. While Case and coworkers argue that these EPCs do not directly differentiate into ECs, they agree that these hematopoietic-derived cells contribute to vascular repair and do so in an indirect manner.33–37 EPCs can be recruited to injured or angiogenic sites and secrete regulatory cytokines that promote vessel homeostasis and vascular repair. Whether these cells incorporate and differentiate directly into ECs has been highly dependent on the vascular bed. In this case, the retinal vasculature may be particularly unique and the contribution of the circulating cells may be more readily apparent in that the resident retinal vasculature is typically quiescent, making the circulating cell contribution highly apparent.38 Thus, we strongly believe that the degree of EPC incorporation and contribution to neovessels may be influenced by competition between EPCs and the resident ECs during the vascular repair process.
When using animal models, the genetic background of the mouse, the type of vascular injury to be incurred, and the organ system to be challenged can all impact the outcome of EPC studies. For example, EPC contributions to tumor endothelium are highly dependent on tumor type, stage, and therapy.39 The type of tissue injury may also be critical, because the cellular and molecular mechanisms of the neovascularization response may differ greatly. For example, laser occlusion of a vessel to induce ischemia may be very different than the thrombotic occlusion of a cardiac vessel in a patient having a myocardial infarction. Moreover, since the tissue “cues” are different, distinct specific cell subsets may be preferentially recruited. Thus, it is not surprising that conflicting results may ensue depending on the animal, the tissue, and the type of injury.
Bone marrow–derived CD14+ monocytes also demonstrate potential to differentiate into endothelial-like cells.40 Interestingly, although several independent groups have shown a clear development of an endothelial phenotype after selective culture, they have shown that the expression of monocytic antigens persisted following adopting this endothelial phenotype.40,41 Several additional studies confirmed the overlap between ECs and monocyte phenotypes, suggesting that using traditional EC markers such as acLDL uptake, Ulex binding, CD31, CD105 (endoglin), CD144 (vascular endothelial cadherin), VEGFR-2, CD34, and Tie-2 may not be enough to distinguish between ECs and monocytes, and thus may not specifically identify EPCs.42–44 Similarly, another group showed that cultured CD34–CD14+ peripheral blood mononuclear cells express Tie-2, VEGFR-2, CD144, von Willebrand factor (vWF), CD146, CD105, and eNOS.41 Interestingly, another report suggested that CD14+ monocytes, which express low levels of CD34 (undetectable with conventional techniques), may in fact contain the true progenitor cell population, with the greatest regenerative capacity.45
The failure to reliably characterize endothelial progenitors has generated some skepticism and confusion within the field of regenerative medicine. However, a recent study46 and an excellent review by Schatteman et al.47 have suggested that despite phenotypic overlap between various bone marrow–derived or circulating cell types, the endothelial phenotype can best be characterized by three features: eNOS expression, integration into tube-like structures formed by human umbilical vein endothelial cells (HUVECs), and stimulation of tube formation by HUVECs. It is suggested that these criteria might be the best in vitro method of judging the endothelial phenotype, regardless of the presence or absence of particular cell surface antigens. This simplified interpretation is significant, as it acknowledges the limitation of surface markers and affirms the importance of the identification of EPCs according to their potential to acquire functional properties of ECs.
In addition to the use of surface markers to isolate cells, the use of culture conditions can be used to identify EPC populations. Most investigators, out of sheer frustration in obtaining adequate numbers to sufficiently study EPCs in a disease model, use in vitro growth of mononuclear cells. Numerous assays have been developed to plate mononuclear cells in specific culture conditions to make putative EPCs differentiate into ECs,7,8,48,49 expand ex vivo,50,51 and to assay EPCs for colony forming capacity (Fig. 27.3).52 Two major cell types have been shown to emerge out of these mononuclear cell cultures: cells that display a mixed endothelial-monocytic/hematopoietic phenotype,4,53 which are referred to as “endothelial-like” monocytic cells or early EPCs (eEPCs), and cells with high proliferative potential that display typical endothelial characteristics, which are called outgrowth endothelial cells (OECs) or late EPCs.51,54
Both endothelial-like cells and OECs express similar surface markers such as CD31, lectin binding, vWF, and uptake of LDL.4 However, recent studies have shown that “endothelial-like” cells co-express a set of endothelial surface markers4,55,56 and have some characteristics of ECs, but do not form ECs in vivo. Further complicating matters, these cells may change their surface marker expression profile upon ex vivo culture and upon injection in vivo.57 Thus, true EPCs need to form blood vessels in vitro and in vivo.
When considering what is really important in an EPC population, one has to consider the origins of the EPC and how the cells first characterized by Asahara et al. compare to the more recently identified OECs. Asahara et al. used a subset of CD34+ hematopoietic progenitor cells.7,58 They reported that peripheral blood mononuclear cells enriched for CD34+ cells could differentiate into endothelial-like cells following culture on fibronectin in the presence of growth factors. After 7 days in culture, the fraction of cells co-expressing CD34 and VEGFR-2 increased. These cells also expressed other endothelial markers such as CD31 (platelet EC adhesion molecule-1, PECAM-1), tyrosine kinase with immunoglobulin-like and EGF-like domains 2 (Tie-2) and eNOS, incorporated acetylated low-density lipoprotein (acLDL), bound Ulex (UEA-1) lectin, and formed tube-like structures in vitro, supporting the contention that they possess the ability to differentiate into ECs. These findings were corroborated by Shi et al.8
Currently, culture selection involves the growth of peripheral blood mononuclear cells in selective medium and on either fibronectin coated dishes or collagen coated dishes.59,60 The medium typically contains a cocktail of endothelial growth factors, such as VEGF, FGF-2, IGF-1, EGF as well as ascorbic acid and hydrocortisone. The medium is changed regularly (usually every 24–28 hours). It is not surprising that exposing cells to endothelial growth factors and placing them on matrixes that are known to “change” their appearance toward an endothelial phenotype will make them appear more “endothelial-like.” These eEPCs have been most studied to date. eEPCs are those mainly derived from monocytes and do not proliferate long term but begin to gradually die after a few weeks in culture.40,61 These characteristics are not indicative of what this population would do in vivo.
In contrast, late OECs usually begin growing after 2 weeks postisolation, eventually proliferate very rapidly, resemble microvascular ECs (with a cobblestone morphology), and have great proliferative potential62,63 The distinction between these two cell types is reinforced by their expression of different markers. OECs do not express CD1a or CD14 and have a low expression of CD45, the panleukocyte marker.64 The precise origin of the OEC is still in question, yet Yoder’s group believes that the endothelium is the likely source of this progenitor and not the bone marrow.4 The OEC represents one in a million mononuclear cells found in the circulation and thus can only be “identified” once it has grown out in culture.22 However, little has been done with these cells in vivo and they have not been studied in humans as of yet.
Since most early studies of cell therapy in the heart and limbs employed eEPCs, there is abundant evidence that these transplanted cells can enhance revascularization,65 although there is much debate about the precise mechanism of these effects. In contrast, OECs have been minimally studied in vivo.56 Still their higher proliferative potential may be important when a large supply of regenerative cells is required. Therefore, OECs may have great utility as a cellular therapy.47
WHAT CAUSES EPC DYSFUNCTION IN DIABETIC PROGENITOR CELLS?
Much of our focus has been on explaining the migratory dysfunction that diabetic EPCs exhibit, as detailed in Figure 27.4. We have determined that this is due in part to reduced nitric oxide availability. NO generation arises from the guanidine group of l-arginine and is a Nicotinamide adenine dinucleotide phosphate (NADPH)-dependent reaction catalyzed by a family of NOS. Three distinct isoforms of NOS, consisting of endothelial NOS (eNOS), neuronal NOS (nNOS), and inducible NOS (iNOS) are similar in structure and function, utilizing l-arginine, oxygen, and NADPH as substrates and requiring Flavin adenine dinucleotide (FAD), Flavin mononucleotide (FMN), calmodulin, and tetrahydrobiopterin as cofactors. The catalytic mechanism of NOS involves flavin-mediated electron transport from NADPH to the terminal heme, where oxygen is bound and incorporated into NO and citrulline.66 Maintaining an adequate cellular supply of l-arginine is critical for normal vascular function. Deficiencies in l-arginine supply have been strongly implicated in vascular diseases, including diabetes.67 If the supply of l-arginine or any cofactor does not meet the needs of active NOS, NOS becomes “uncoupled” and uses molecular oxygen as a substrate to form O2– instead of NO. An imbalance between l-arginine availability and NOS activity can occur when cellular transport of l-arginine is inhibited,68 or when there is reduced recycling of l-citrulline back to l-arginine, or when an elevated catabolism of l-arginine by arginase exists.69,70
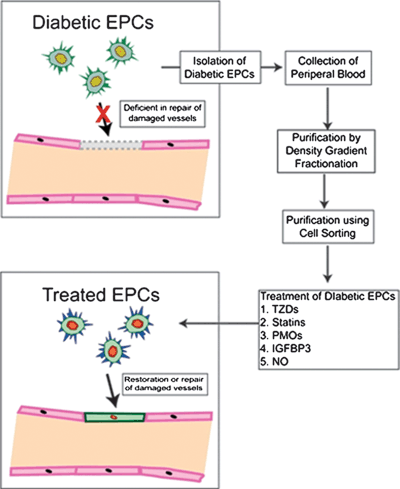
FIGURE 27.4. EPC dysfunction in diabetes. Healthy EPCs have proven ability to repair damaged endothelium by homing to sites of vascular damage and tissue ischemia. EPCs from diabetic donors lack this reparative ability due to inherent cytoskeletal defects and/or impaired signaling within defined transduction pathways. Ex vivo pharmacological manipulation of diabetic EPCs can restore this diabetic defects, improving their reparative ability prior to autologous therapeutic transfer.
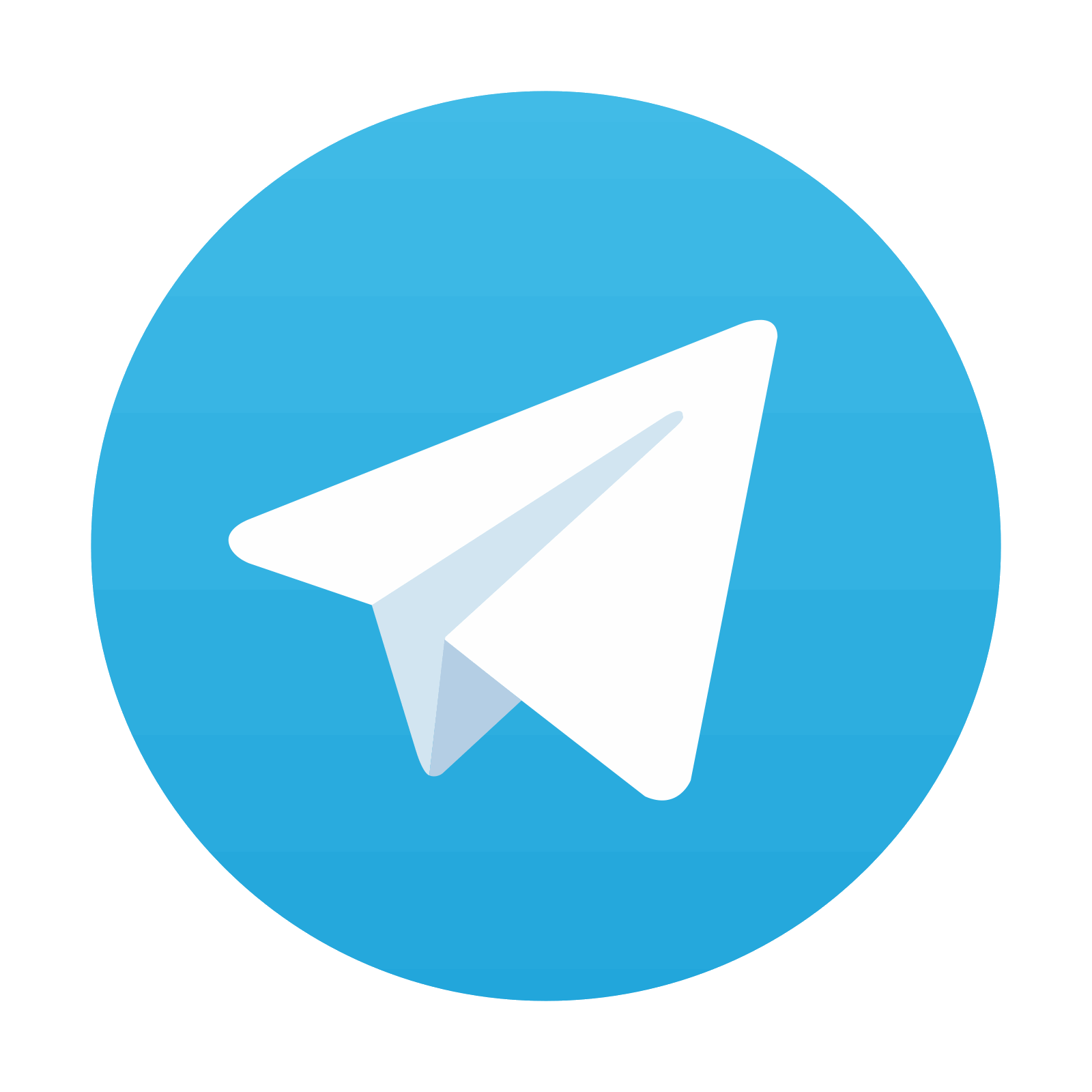
Stay updated, free articles. Join our Telegram channel
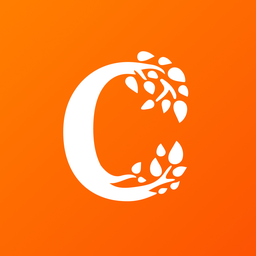
Full access? Get Clinical Tree
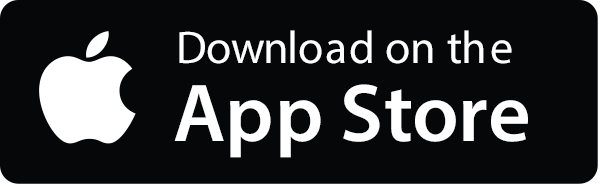
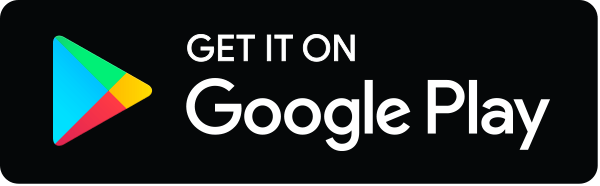