Pathologic neovascularization occurs in various ocular tissues, including the cornea, iris, retina, and choroid
Tissue | Disease/Trauma |
---|---|
Cornea | Contact lens injury |
Trauma/alkali injury | |
Infectious keratitis (trachoma) | |
Iris | Enucleation (i.e., retinoblastoma) |
Central retinal vein occlusion | |
Diabetes mellitus or other forms of RNV | |
Retina | Proliferative diabetic retinopathy/other diabetic complications |
Retinopathy of prematurity | |
Sickle cell retinopathy | |
Central retinal vein occlusion | |
Branch retinal vein occlusion | |
Rare disorders (i.e., Eales disease) | |
Choroid | Age-related macular degeneration |
Myopia |
Though the underlying pathogenesis of these vasoproliferative disorders of the retina can differ, their final outcomes can be loosely divided into retinal neovascularization (RNV) and choroidal neovascularization (for review see Ref. 5). RNV is caused by the growth of blood vessels along the inner surface of the retina and can be a complicating factor in retinal ischemic events, such as occurs in diabetic retinopathy and ROP. In diabetic retinopathy, hyperglycemia leads to closure of retinal capillaries and decreased retinal perfusion. In ROP, stunting of the retinal vascular network results in insufficient blood flow to the peripheral retina. Ultimately, these ischemic events result in the activation of hypoxia inducible factor 1 (HIF-1),6 which induces expression of vascular endothelial growth factor (VEGF) and other hypoxia-stimulated genes.7 CNV involves the growth of blood vessels below the retinal pigment epithelium (RPE) and most commonly consists of proliferation from the choroidal plexus. However, blood vessels can occasionally sprout from the deep capillaries of the retina, a condition termed retinal angiomatous proliferation. In AMD, the etiology of angiogenic stimulation is less well understood (for reviews see Refs. 8 and 9) but appears to depend on the breakdown of Bruch membrane at the macula and, in the severe form, invasion of blood vessels through the RPE into the subretinal space.
CURRENT TREATMENT MODALITIES
Current treatments for AMD and diabetic retinopathy include laser photocoagulation and photodynamic therapy, both of which cauterize new blood vessels by scar tissue formation or destruction of tissue. However, this short-term treatment fails to address the underlying pathogenesis of blood vessel growth, often leading to a high incidence of disease recurrence. Almost half of AMD patients have been reported to exhibit persistent and recurrent neovascularization within 3 years of laser treatment.10 In fact, laser treatment has been found to be detrimental compared to no treatment in AMD patients with occult CNV, a condition in which leakage from blood vessels occurs in punctate areas in multiple distinct regions.11 Such restrictions on current treatment highlight the need for the development of novel therapeutics to inhibit neovascularization.
While small molecule drugs are attractive due to increased stability and cost-effective large-scale production, preclinical data indicate that recombinant antiangiogenic proteins have fewer side effects and may be better suited for therapy.12 Clinical trials over the past few years have begun to assess the efficacy of drugs injected into the vitreous of the eye and, as of 2008, more than 60 trials for the treatment of AMD were recruiting patients.13 Currently, only two of these regimens have been approved for the treatment of wet AMD. In December 2004, the Food and Drug Administration (FDA) approved pegaptanib sodium (Macugen), an mRNA aptamer against a single VEGF isoform, for the treatment of wet AMD. However, only a subset of patients demonstrated improvements in visual acuity, leading to a subsequent decrease in popular use.13 In 2006, the FDA approved ranibizumab (Lucentis), a modified humanized monoclonal antibody fragment against all VEGF-A isoforms. Lucentis has shown efficacy in halting the progression of disease and, in some cases, reversing vision loss.13 Many of the therapies used to treat wet AMD are being evaluated for efficacy in other neovascular disorders.
LIMITATIONS OF CURRENT TREATMENTS
Aberrant blood vessel growth is critical to the pathogenesis of proliferative diabetic retinopathy and AMD. The chronic nature of both these disorders requires that inhibition of neovascularization be sustained over a long period of time, while the short half-life of many antiangiogenic molecules, such as pegaptanib and ranibizumab, necessitates repeat injection.14 Though oral administration of antiangiogenic molecules has proven effective in inhibiting ocular neovascularization,15 there are concerns over the consequences of long-term systemic exposure to antiangiogenic molecules16; for example, VEGF neutralizing molecules could disrupt the normal effects of VEGF on processes such as wound healing and endometriosis. Local administration of these molecules relies on repeat ocular injection, typically intravitreal, which brings with it several complications, such as endophthalmitis and retinal detachment, both of which have the potential for blinding.16 While the risk with each injection is low, the cumulative risk over many years may be substantial.
Gene therapy has the potential to improve current therapies.17 Long-term expression of the therapeutic protein would theoretically reduce administration of the treatment to a single event. By targeting specific tissues, and regulating gene expression, gene therapy allows for more precise control over exposure and dosing of the drug. The ability to deliver multiple transgenes could allow for the targeting of multiple steps or multiple pathways in the angiogenic process. In addition, recombinant proteins can be expensive to produce, so expression from gene therapy vectors has the potential to reduce cost. While there are many advantages associated with a gene therapy approach, a number of possible complications still limit application in the clinic, including immune reactions, integration into the host genome, cytotoxicity, and efficacy of transfection.
OCULAR TISSUE AS A TARGET FOR GENE THERAPY
The eye is uniquely suited to overcome barriers previously reported in gene therapy clinical trials. The eye is an immune privileged tissue and so injection does not evoke the normal humoral immune response that can preclude treatment with some vectors, especially viruses.18 For example, injection of a normally immunogenic adenovirus into the subretinal space resulted in little systemic immune response, which could in turn allow for long-term transgene expression.19 Intraocular injections primarily transfect local tissue with little diffusion of gene products into the systemic circulation. Ocular disorders typically occur bilaterally and the contralateral eye may act as an internal control. In addition, the translucency of the anterior portion of the eye allows for noninvasive monitoring of efficacy and toxicity of treatment.
Gene therapy approaches have demonstrated a high efficacy in the rescue of animal models of disease, ranging from single loss-of-function mutations in retinal degeneration models to complex acquired disorders (for review see Ref. 20). Gene replacement has proven effective in animal models of single gene disorders such as Leber congenital amaurosis (LCA)21,22 and retinitis pigmentosa.23–25 The expression of growth factors and signaling inhibitors has shown efficacy in preventing the pathogenic sequela in animal models of complex disorders such as retinal26–28 and choroidal27–31 neovascularization, experimental autoimmune uveoretinitis,32 and experimental glaucoma.33
Recently, gene therapy clinical trials have shown positive outcomes in ocular disease34 (Table 26.2). Neurotech Pharmaceuticals demonstrated that an intravitreal implant containing encapsulated cells transfected to secrete ciliary neurotrophic factor was safe for use in retinitis pigmentosa patients, with a subset of those treated showing improvement in visual acuity.35 Virus-based clinical trials include retrovirus delivery of a dominant negative cyclin G1 construct for the treatment of superficial corneal opacity/corneal scarring,36 adenovirus-based delivery of pigment epithelium–derived factor (PEDF) for the treatment of wet AMD37 (see Box 26.1), adenovirus delivery of herpes simplex virus thymidine kinase for the treatment of refractory retinoblastoma,38 and adeno-associated virus (AAV) delivery of the RPE65 gene for rescue of LCA.39–41 No dose-limiting toxicities were observed after adenoviral injection in the retinoblastoma patients, with seven of the patients treated at the highest dose exhibiting resolution of the tumor.38 Results from three different Phase 1 trials have shown short-term safety of the AAV viral vector in LCA patients,39–41 with one study reporting modest improvement in visual acuity in all subjects.39 In the latter study, further recovery of visual acuity was noted at the 1-year time point. Together, these trials have established gene therapy as a clinically viable approach in the treatment of ocular disease.
Table 26.2.
Ocular gene therapy clinical trials
Therapy | Mechanism | Phase | Disease |
---|---|---|---|
AdV-Tk | HSV thymidine kinase expression with intravenously delivered ganciclovir | Phase 1 | Refractive retinoblastoma |
ADGVPEDF.11D | Adenovirus expressing PEDF | Phase 1 | Wet AMD |
Mx-dnG1 | Retrovirus expressing dominant negative cyclin G1 | Phase 1/2 | Adjunctive therapy for superficial corneal opacity/corneal scarring |
NT-501 | Implant of encapsulated human NTC-201 cells releasing CNTF | Phase 2 | Retinitis pigmentosa |
Phase 2 | Visual impairment from AMD | ||
rAAV2-CB-hRPE65 | AAV expressing RPE65 | Phase 1/2 | Leber congenital amaurosis Type 2 |
AAV2-sFLT01 | AAV expressing soluble FLT-1 | Phase 1 | Wet AMD |
BOX 26.1 Gene Therapy Phase 1 Clinical Trial: Adenovirus Mediated Expression of PEDF for the Treatment of Neovascular AMD
AMD is the leading cause of blindness in older adults in the United States,3 with increasing incidence as the population ages. In 2006, Campochiaro et al.37 published the results of a Phase 1 clinical trial for the treatment of AMD, the first gene therapy clinical trial for ocular angiogenesis. This study tests the use of a serotype 5 adenovirus (Ad5) expressing human pigment epithelium–derived factor (PEDF) (AdPEDF.11). Previously, studies in animal models have shown that injection of an earlier generation virus, AdPEDF.10, into the vitreous expresses high levels of PEDF protein 5 days postinjection. AdPEDF.11 has been shown to inhibit VEGF-induced CNV, laser-induced CNV, and hypoxia-induced RNV.28 The inhibitory effect of AdPEDF.11 on laser-induced CNV was found to be over twofold higher when injected into the subretinal space.28 Importantly, for the treatment of AMD patients, intravitreal injection of AdPEDF.11 was shown to cause regression of established laser-induced CNV.152 In a pig model of laser-induced CNV, 109 particle units (PU) of AdPEDF.11 injected intravitreally caused a 38% reduction in neovascularization after 10 days.134
The initial Phase 1 trial was a dose escalation study, ranging from 106 to 109.5 PU injected into the vitreous to evaluate potential toxicity of the vector. Previously, doses of 1010 PU or higher were shown to cause an increasingly severe inflammatory response in nonhuman primates.162 Patients were monitored in 13 visits over a 12-month follow-up period. While there was mild transient inflammation in 25% of patients, no severe inflammation was noted, even at the highest dose tested. This agrees with previous Phase 1 results using adenovirus in which no toxicities were observed other than mild, controlled inflammation even up to 1010 PU, a higher dose than used with AdPEDF.11.38 The increase in intraocular pressure observed in 6 of the 28 subjects was restored to normal using topical treatment. Serum adenoviral cultures were inconsistent, but remained low over time, and urine and sputum cultures were negative.
Fluorescein angiography was used to evaluate the size and leakage of the neovascular lesion. After 6 months, in the low-dose group (106–107.5 PU) 50% of patients showed stabilization or improvement in CNV lesion size and in the high-dose group (108–109.5 PU) 71% of patients showed stabilization or improvement in lesion size. While the median lesion size increased by 0.5 disk areas at 6 months and 1.0 disk areas at 12 months in the low-dose group, there was no increase in lesion size at either 6 or 12 months in the high-dose group. This appeared to correlate with functional tests in which patients in the high-dose group showed stable visual acuity over the 12-month period, whereas the low-dose group appeared to show deterioration of visual acuity at 6 and 12 months. Thus far, Phase 1 results using AdPEDF.11 indicate that at doses higher than 106 PU, long-term treatment of disease progression out to at least 12 months may be achieved. At this point, however, it is unclear if this is due to continued PEDF production, or the sustained effects of initial high PEDF expression that later turns off (AdPEDF.11 uses the CMV promoter, whose expression has been shown to decrease to undetectable levels after 90 days in the eye144). Taken with results from other gene therapy trials, there is strong support for the continuation of gene therapy strategies for the long-term treatment of ocular neovascular disorders such as AMD.
THERAPY TARGETS FOR OCULAR NEOVASCULARIZATION
Gene therapy strategies for the treatment of single gene disorders such as LCA, in which the genetic defect leading to pathology has been determined, are fairly straightforward. Development of potential therapeutic molecules in CNV and RNV, in which the molecular basis of the disease is more complex and less understood, has proven challenging.
Vascular Endothelial Growth Factor
Multiple lines of evidence suggest that VEGF plays a central role in the pathogenesis of ocular neovascularization. Patients with ischemic retinal disease and progression to RNV have been shown to exhibit a concurrent increase in VEGF in the ocular fluid.13,42 In fact, VEGF has been shown to be both necessary and sufficient for induction of experimental neovascularization.43–47 Drugs approved for the treatment of ocular angiogenesis, such as Lucentis, modulate VEGF, and anti-VEGF molecules delivered to animal models have shown significant reductions in both CNV and RNV.48 These same molecules have shown comparable therapeutic efficiencies when delivered via a gene therapy approach. For example, a soluble VEGF receptor protein shown to prevent neovascularization44 also does so when a transgene expressing the same protein is delivered.49
While current candidate molecules used in gene therapy experiments in the eye have focused primarily on VEGF inhibition, other inhibitors of angiogenesis have been identified. These include secreted angiostatic proteins, soluble receptors for proangiogenic factors, and complement inhibitors (for a review see Refs. 50 and 51). The relative efficacy and potency of these proteins are still under investigation.52
Secreted Angiostatic Factors
Secreted angiostatic factors are derived from naturally occurring proteins and therefore have the potential for a decreased immune response. One such protein, PEDF, was identified as a potent angiogenic inhibitor in the eye, with the ability to inhibit multiple proangiogenic factors.53 PEDF is naturally produced by a number of different ocular cell types in humans, including the RPE and photoreceptors,54 and loss of expression could at least partially contribute to disease. Downregulation of PEDF is known to occur in response to hypoxia53 and expression of PEDF has been shown to be inversely correlated with age,55 implicating it as a possible mediator of angiogenesis in AMD. PEDF has been reported to have neuroprotective properties56 and has been shown to delay photoreceptor loss in a mouse model of retinal degeneration,57 further enhancing its consideration as a therapeutic candidate.
Other endogenously secreted antiangiogenic molecules that have specific action against proliferating endothelial cells have been identified.17 Angiostatin, a cleavage product of plasminogen,58 has the ability to block capillary cell proliferation.59 Endostatin is a proteolytic fragment of collagen XIII shown to inhibit VEGF-induced endothelial cell migration.60 Additionally, endostatin has been shown to be inversely correlated with the severity of neovascularization in diabetic retinopathy.61 In order for blood vessels to invade the retina in AMD, the barrier of the Bruch membrane must first be degraded. This is largely mediated by metalloproteinases (MMPs). Thus, inhibitors of MMPs, such as TIMP-3, have antiangiogenic properties.62
There is some evidence that indicates that angiostatic soluble factors work to inhibit angiogenesis with roughly the same efficiencies. In one study, AAV vectors were used to examine the efficacies of three secreted factors: PEDF, endostatin, and TIMP-3.26 In an animal model of ocular neovascularization, all three molecules were able to significantly decrease the number of proliferating endothelial cells with efficacies ranging from 75% to 85%, though TIMP-3 appeared to be the least effective in this study.26 In a separate study, AAV expression of PEDF and angiostatin inhibited the formation of neovascular nuclei by 74% and 78%, respectively.63 While their potencies appear roughly comparable, it is worth noting that these proteins each inhibit angiogenesis via different mechanisms, so targeting multiple pathways by delivering multiple transgenes may allow for even greater antiangiogenic efficiency.
Soluble Receptors
Soluble receptors have also shown potency in inhibiting angiogenic signaling. The soluble form of the VEGF receptor has been shown to bind VEGF and block signaling.64 Novel chimeric molecules of this receptor have been generated and shown to be effective in inhibiting neovascularization.65 The Ang2 protein has been shown to be upregulated in surgically removed CNV membranes,66 and the soluble form of its receptor Tie2 (ExTek) is shown to inhibit angiogenesis.27,67
A direct method of VEGF signaling inhibition is the knockdown of VEGF protein production itself, making it an ideal target for antisense RNA-mediated knockdown.68 Two approaches have been used to deliver silencing RNA in the eye: delivery of small interfering siRNA against VEGF69,70–71 and VEGF receptor 1.72 Additionally, siRNA against HIF-1α, a hypoxia inducible factor that upregulates VEGF expression, has also been shown to be effective against in vivo angiogenesis.73 In a recent report, siRNA activation of Toll-like receptor 3 (TLR3) led to inhibition of angiogenesis in two models of ocular neovascularization, independent of RNA sequence.74 This implied that general mechanism of siRNA neovascular inhibition raises concerns over the exact mechanism of antiangiogenesis using intravitreal injection of naked anti-VEGF siRNA.70,72 Such concerns could be addressed by expression of the antisense RNA within the cell using a gene therapy approach, thereby avoiding interaction of the siRNA with extracellular receptors. A short hairpin RNA (shRNA) targeting VEGF and expressed from an adenoviral vector has been shown to inhibit CNV,75 thereby validating this approach.
The activation of endogenous antiangiogenic genes was recently shown to be an efficient inhibitor of ocular neovascularization.76 In one study, AAV delivery of a zinc-finger transcription factor, engineered to upregulate PEDF gene expression, was able to reduce CNV after laser induction for up to 3 months.76 The authors point to the use of this technology as a potential prophylactic treatment for at-risk AMD patients who have not yet developed neovascularization. Conversely, suppression of endogenous angiogenic factors would also inhibit neovascularization. The expression of GA-binding protein (GABP), a transcription factor that regulates the expression of VEGF and roundabout4 (Robo4), has been shown to decrease VEGF and Robo2 levels in vivo and inhibit angiogenesis.77
Complement Inhibitors
Recent work has shown that induction of the membrane attack complex (MAC), the final step in the complement cascade, is a critical mediator of laser-induced CNV.78 Importantly, CD59, an inhibitor of MAC deposition, was found to be downregulated in laser-induced CNV, and CD59 null transgenics were more susceptible to CNV induction.79 The potential for CD59 to inhibit CNV was confirmed by inhibition of MAC deposition and attenuation of laser-induced CNV after administration of a soluble CD59.79 Adenovirus-mediated expression of membrane-bound human CD59 has been shown to prevent MAC deposition on the cornea and RPE of a murine ex vivo humanized model of AMD80; such an approach could further restrict the expression of a potent complement inhibitor to the relevant tissue. Polymorphisms in the complement factor H gene, an inhibitor of the alternative complement pathway, have been found to be associated with AMD.81 Laser-induced CNV has been shown to be reduced by both recombinant factor H82 and the protein thioredoxin-1,83 which acts through an interaction with factor H, providing novel therapeutic avenues for treatment.
Other Molecules
Other proteins have been isolated and found to inhibit angiogenesis in vivo. For example, von Hippel-Lindau (VHL) protein, a mutant form of which causes the hereditary cancer syndrome von Hippel-Lindau, has been shown to decrease basal levels of VEGF transcripts in vitro when overexpressed in various cell types, including the RPE.84 Adenovirus delivery of VHL transgene decreased VEGF expression and suppressed neovascularization in a nonhuman primate model of laser-induced branch retinal vein occlusion in vivo.84 The anti-VEGF intraceptor gene (Flt23K) is a recombinant hybrid protein consisting of VEGFR-1/Flt-1 domains 2 and 3 (which bind VEGF) fused to the KDEL endoplasmic reticulum (ER) retention signal. KDEL binds ER proteins and prevents their secretion. Thus, upon expression, the anti-VEGF intraceptor binds VEGF intracellularly and sequesters it within the ER and has been shown to decrease ocular angiogenesis.85
A variety of molecules have been shown to inhibit angiogenesis in ocular tissue, ranging from soluble factors that bind VEGF directly to transmembrane molecules that inhibit neovascular initiation (Fig. 26.1). Many of these molecules have been tested via a gene therapy approach and proven successful in a wide array of animal models. A number of different gene therapy vectors have been tested in their ability to deliver these antiangiogenic factors and prevent neovascularization in vivo.
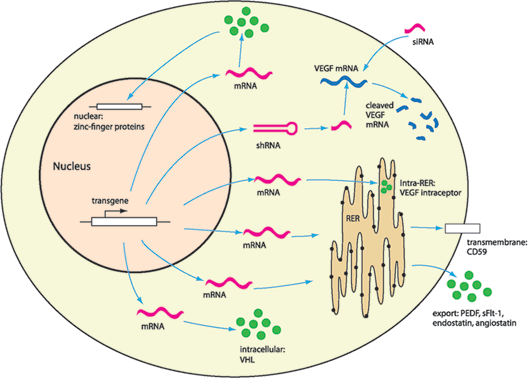
FIGURE 26.1. Gene therapy–mediated transgene expression targets molecules intracellularly and extracellularly. Targeted degradation of mRNA corresponding to angiogenic proteins, such as VEGF, has been demonstrated using shRNA and siRNA. Transgenic recombinant proteins can inhibit neovascularization by a variety of mechanisms, including sequestering VEGF protein in the RER (VEGF intraceptor), inhibition of MAC deposition on the cell surface (CD59), modulation of intracellular pathways (VHL), activation or inhibition of gene transcription (zinc-finger proteins), or secretion of antiangiogenic proteins (PEDF, sFlt-1). Gene therapy allows for inhibition of angiogenesis at many different locations by the expression of multiple transgenes. RER, rough endoplasmic reticulum; VEGF, vascular endothelial growth factor; PEDF, pigment epithelium–derived factor; VHL, von Hippel-Lindau protein.
GENE DELIVERY VECTORS
There are many approaches to gene delivery in the eye that have shown efficacy in the rescue of animal models of disease. A number of different criteria must be considered when choosing an ideal vector for use in the clinic. Such factors include either nonspecific or site-specific integration in the host genome, lack of inflammation and immune response, specific transduction of target cells, large carrying capacity, long-term expression, and high transfection efficiency (reviewed in Ref. 86). Transgene delivery can occur through a variety of mechanisms but involves targeting of the vector to a specific cell type, binding and uptake at the cell surface, escape from the endosome, release of DNA, and movement of DNA into the nucleus for transcription (Fig. 26.2). Despite differences in mechanism, a number of vectors have shown efficacy as gene delivery vehicles for the rescue of ocular neovascularization in animal models. For an overview of vectors, see Table 26.3.
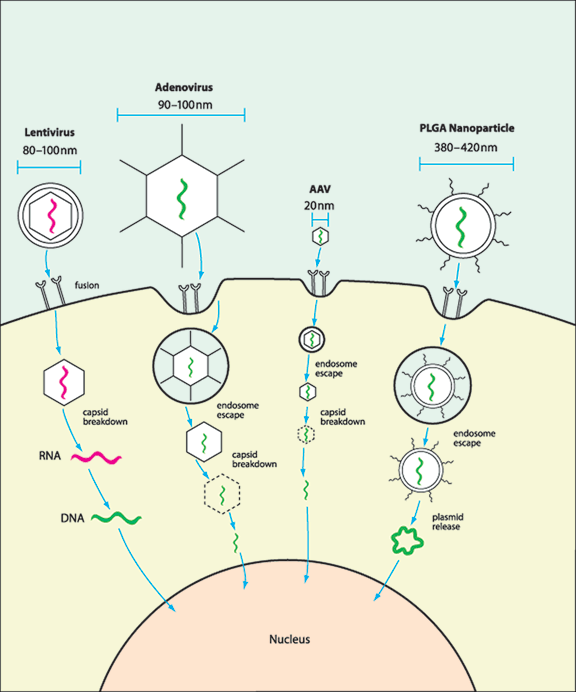
FIGURE 26.2. Vectors have different mechanisms of nuclear transgene delivery. While many vectors have been shown to transfect ocular tissue, only a few have demonstrated targeted transfection and inhibition of angiogenesis. Lentivirus, a member of the retrovirus family, fuses with the cell surface, eventually releasing its RNA genome, which is subsequently reverse-transcribed into DNA, transported to the nucleus, and integrated into the host genome. Adenovirus triggers endocytosis via interaction between the fiber protein and cell receptors. Within the endosome, capsid degradation is initiated, leading to endosomal escape and eventually release of viral DNA, which can then be transported to the nucleus. AAV entry proceeds by a similar mechanism binding the cell surface, triggering endocytosis and escape, and eventual trafficking to the nucleus. Within the nucleus, AAV must then mature the genome from single- to double-stranded DNA. PLGA nanoparticles are thought to bind receptors on the cell surface, trigger endocytosis and subsequent escape, release plasmid DNA into the cytoplasm, and finally transport of the DNA into the nucleus.
Table 26.3.
Features of gene therapy vectors
Retrovirus | Adenovirus | AAV | Nonviral Peptide | Nonviral Lipid | Physical | |
---|---|---|---|---|---|---|
Transgene | RNA | dsDNA | ssDNA | dsDNA | dsDNA | dsDNA |
Duration of expression | Long | Short | Long | Short | Short | Short |
Delay in transgene expression | 1 wk | 2 d | 1–4 wk | 2 d | 2 d | 2 d |
Average transgene cloning capacity | 8 kB | 8–36 kB | 5 kB | High | High | High |
Transfection efficiency | High | High | High | Low | Low | Medium |
Primary cell target | RPE | Many | Many | RPE | RPE | RPE |
Ease of production | High | High | Low | High | High | High |
Immune reaction | Low | Low–high | Low | Low | Low | Low |
Integration | High | Low | Possible | Low | Low | Low |
Cytotoxicity | Low | Low | Low | Low | High | High |
Viruses are a common vector for ocular gene delivery, primarily because of their high transfection efficiency. In the eye, lentiviruses (and other members of the retrovirus family), adenovirus, and AAV have all been explored. In general, the most commonly used serotypes of all three viruses show a high tropism for the RPE upon injection into the subretinal space, with little to no transduction of other cell types in the retina. In some cases, this tropism can be overcome by a process called pseudotyping. Pseudotyping is the exchange or conjugation of one of the wild-type viral coat proteins with a protein whose receptor is known to be abundantly expressed on the target cell. This strategy has proved at least partially successful in altering the tropism of all the viruses listed above (Fig. 26.3).
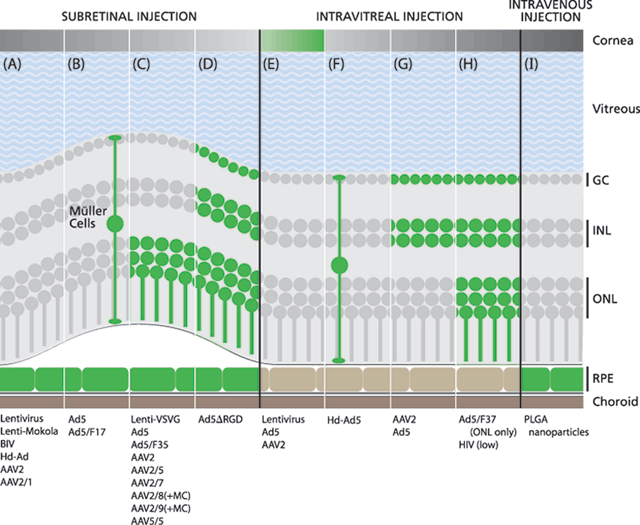
FIGURE 26.3. Pseudotyping and functionalization of gene therapy vectors can alter tissue tropism. While some vectors are consistently reported to show the same transfection patterns, others exhibit variable tropism potentially influenced by outside factors, such as transgene detection sensitivity, viral titer, and duration of incubation. (A) Transduction of primarily RPE after subretinal (SR) delivery of lentivirus,88 lenti-Mokola,92 BIV,93 Hd-Ad,94 AAV2, and AAV2/1.92 (B) Transduction of RPE and Müller cells after SR delivery of Ad599,101 and Ad5/F17.101 (C) Transduction of RPE and photoreceptors after SR delivery of lenti-VSVG,92 Ad5,84 Ad5/F35 (and Müller cells),99 AAV2,31,92,129,151 AAV2/5,92,111 AAV2/7,111 AAV2/8 (and Müller cells),111 AAV2/9 (and Müller cells),111 and AAV5/5.92 (D) Transduction of RPE, photoreceptors, bipolar cells, and ganglion cells after SR delivery of Ad5∆RGD.101 (E) Transduction of the corneal epithelium after intravitreal (IV) delivery of lentivirus,88 Ad5,49,69,160 and AAV2.129 (F) Transduction of Müller cells after IV delivery of Hd-Ad5.109 (G) Transduction of ganglion cells after IV delivery of AAV231,49,92 and Ad5.159 (H) Low transfection levels of ganglion cells, bipolar cells, and photoreceptors after IV delivery of HIV.153 Transduction of photoreceptors after IV delivery of Ad5/F37.100 (I) PLGA nanoparticles transfect the RPE after intravenous delivery.85 AAV2/5, AAV2/1, and AAV5/5 show little transfection after IV delivery.92 GC, ganglion cells; INL, inner nuclear layer; ONL, outer nuclear layer; RPE, retinal pigment epithelium.
Lentiviruses
In the postmitotic retina, lentiviruses are able to deliver genes to the RPE87 but are inefficient at infecting adult photoreceptors.88 When injected into the vitreous, lentiviruses have been reported to efficiently infect the corneal endothelium.89 Lentiviruses pseudotyped with Equine Infections Anemia Virus (EIAV) or vesicular stomatitis virus glycoprotein (VSVG) coat proteins show sporadic infection of photoreceptors,90,91–92 but lentiviruses pseudotyped with the Mokola envelope display tropism exclusively for the RPE.92 There is some interest in the use of viral constructs that do not typically infect human cells, such as bovine immunodeficiency virus (BIV), which has been shown to stably infect the RPE after virus delivery into the subretinal space.93 However, BIV still showed a lower transduction efficiency compared to similar experiments with Hd-Ad.94 Lentiviruses have a cloning capacity of only approximately 7 kb and integrate into the host genome as part of their life cycle, which can lead to insertional mutagenesis and oncogenicity. Such an event led to the development of leukemia following ex vivo administration of the Moloney murine leukemia retrovirus in a clinical trial for SCID-X1.95 Oxford Biomedica is currently developing an angiostatin and endostatin expressing lentivirus vector for infection of RPE cells as a potential treatment of AMD and proliferative diabetic retinopathy.
Adenovirus
Unlike lentivirus, adenovirus typically remains episomal in the nucleus of infected cells,96 decreasing concerns over virus integration. However, integration has been reported at very high virus titers.97 The necessity for integration to achieve long-term gene expression may not be relevant in the eye, in which cells are mostly postmitotic and therefore loss of episomes during cell division is not a concern. Commonly used adenovirus vectors, such as serotypes 2 and 5, primarily infect the RPE in adult retina.98 Altering the coat proteins of adenovirus serotype 5 (Ad5) either by fiber replacement using a serotype 35 (Ad5/F35)99 or 37 fiber (Ad5/F37)100 or by deletion of an RGD domain in the penton base (AdΔRGD)101 can allow for the efficient transduction of photoreceptors. Ad5/F35 virus with additional modification of an RGD domain to the knob portion (Ad5/F35 RGD) has also been shown to have increased duration of transgene expression compared to Ad5.102 Concerns over the safety of adenovirus vectors were raised after the death of a patient in whom the virus was shown to trigger the immune system after high-dose injection into the liver.103 However, two clinical trials using adenovirus vectors in the eye have reported no adverse events or limiting toxicities other than mild inflammation that was easily managed.37,38
Adenoviruses have been developed to overcome a particular hurdle of viral gene therapy: that of limited cloning capacity. First-generation adenovirus only had a cloning capacity of approximately 7 kb, but the development of a helper-dependent (Hd) adenovirus has expanded that capacity to approximately 36 kb.104,105–106 This allows for the incorporation of large upstream regulatory elements as well as the inclusion of multiple transgenes. Hd adenoviruses (Hd-Ad) contain only the inverted terminal repeats and packaging signal needed for replication and viral packaging. In first-generation adenovirus studies, the immune response to small amounts of viral gene expression was found to limit the duration of transgene expression.107 However, Hd-Ad vectors have shown increased longevity of expression, up to 1 year, the longest time period tested.108,109 Compared to first-generation adenovirus, a Hd-Ad expressing PEDF showed a substantially reduced cytokine/chemokine gene expression and inflammatory cell infiltrate, as well as a much longer duration of expression.110
Adeno-associated Virus
AAV is much smaller than adenovirus and has a cloning capacity of only approximately 4.8 kb. Like adenovirus serotype 5, the commonly used AAV serotype 2 primarily infects RPE. Pseudotyping of AAV2, by replacement of capsid proteins with those of other AAV serotypes, has proven effective in increasing infection of other tissues. For example, AAV2/8 and AAV2/9 transfect Müller cells and photoreceptors,111 whereas AAV2/5, AAV2/7, and AAV5/5 have significantly increased photoreceptor transduction.92,111 AAV is largely considered to be the least immunogenic viral vector and so is well suited for long-term therapy (reviewed in Refs. 112 and 113). It has been shown to exhibit long-term expression and rescue in animal models of LCA.114 While inflammatory responses, neutralizing antibodies, and RPE atrophy have been reported after AAV delivery to the subretinal space of nonhuman primates,115 after 1 year no adverse effects or toxicities have been documented in the clinical trial performed using AAV for the treatment of LCA. Wild-type AAV naturally recombines at a specific site in the human genome called AAVS1 on chromosome 19.116 The rep protein that mediates recombination is deleted in recombinant AAV (rAAV) to increase the cloning capacity and, though rAAV remains primarily episomal, it has been shown to integrate randomly in human cells.117 There is some evidence of AAV insertional mutagenesis in a mouse model of mucopolysaccharidosis type VII in which many of the animals developed hepatocellular carcinoma118; however, no such events have been documented in other animal models or in the AAV clinical trials for LCA.
Nonviral Gene Therapy
Nonviral gene therapy has the potential for decreased immune responses in the eye, in which even a very small amount of inflammation can exacerbate disease. Many strategies have been employed in the development of nonviral ocular gene therapy (for review see Ref. 119). However, in general, nonviral vectors have had low transduction efficiencies in the eye and there have been few examples of successful rescue of disease models. Nonviral strategies can be loosely grouped into chemical, including cationic lipids and peptides, and physical methods of delivery. Cationic lipids have been shown to cross the blood-retinal barrier and selectively transfect all cells in the eye via a rhodopsin promoter.120–122 Subretinal delivery of Lipofectamine 2000 and NeuroPorter has shown efficient RPE delivery, although Lipofectamine demonstrated substantial toxicity.123 Lipofectamine has been shown to successfully deliver antiangiogenic siRNA vectors in vivo when injected into the vitreous73 while another lipoplex-based transfection reagent, FuGENE 6, has been used to deliver an angiostatic transcription factor to the cornea after subscleral injection.77
The PEGylated polylysine cationic peptide PEG-CK30 compacted DNA into elongated nanoparticles and has been shown to transfect many cell types in the retina at high levels.124 PEG-CK30 is currently in clinical trials for gene delivery to the lungs of cystic fibrosis patients.125 Recently, PEG-CK30 has been shown to partially rescue the peripherin/rds (slow degeneration) mouse model of retinitis pigmentosa.126 Various mechanical approaches, such as electroporation127 and electron avalanche transfection,128 have shown efficacy in gene delivery, but are currently too toxic for clinical application.
One study recently showed evidence for nonviral gene therapy in the treatment of the laser-induced CNV model.85 In this study, poly(lactic-co-glycolic acid) (PLGA) nanospheres, loaded with an anti-VEGF intraceptor gene (Flt23K), were functionalized with RGD and transferrin and shown to transfect the RPE when injected intravenously into the tail vein. The nanoparticles were shown to selectively transduce the laser-treated eye and had low efficiency of delivery to tissue outside the CNV lesion, perhaps overcoming concerns of systemic expression in intravenous delivery.
A number of different vectors, both viral and nonviral, have been designed for gene delivery to ocular tissue. While these vectors have been shown to demonstrate a wide range of tropism, tissue transduction also depends on the location of delivery and the cell types primarily exposed.
MODE OF DELIVERY AND THE TARGET CELL
The eye is unique in that (a) it is easily accessible for local administration of therapeutic genes and (b) isolation of the ocular tissues via the blood-retinal barrier allows for more targeted delivery of gene therapy vectors. Varying the location of administration can further restrict exposure to gene therapy vectors to a specific cell population. Additionally, current vectors not only transduce different cell types (as seen above) but also differ in their ability to penetrate ocular tissue. There are four different sites of injection that have shown efficacy in rescuing animal models of ocular neovascularization: the subretinal space, the vitreous, the periocular tissue, and the vasculature (including intramuscular and intravenous injection). The ideal mode of delivery depends on (a) the therapeutic factor being expressed, (b) the target tissue, and (c) the vector used for delivery.
Subretinal and intravitreal administration of the same vector can result in transfection of different cell types. In one study, upon injection into the vitreous, AAV2 was found to transfect many cells in the retina, primarily ganglion cells (GC) and the inner nuclear layer (INL), whereas in the same study, adenovirus 5 (Ad5) was shown to infect cells of the corneal endothelium, iris pigment epithelium, and ciliary epithelium.49 Interestingly, though they transfected very different cell types, the two vectors had highly comparable levels of RNV reduction in an ROP model.49 Although adenovirus infected cells were relatively farther away from the site of neovascularization, the soluble factor appeared able to diffuse across the vitreous at levels sufficient to inhibit blood vessel growth. Similarly, AAV expressing sFlt-1 delivered into the subretinal space at the equator of the eye was able to inhibit laser-induced neovascularization at the optic nerve, indicating that soluble factors are able to diffuse to the site of neovascularization even if not expressed proximal to the injury site.129 While diffusion can lessen the importance of the injection location, the mode of delivery can also enhance the potency of the vector. For example, in a laser-induced CNV model, rescue using a PEDF expressing adenovirus increased from 33% to 70% after intravitreal versus subretinal injection.28 The benefits of subretinal injection are not always clear; for example, AAV expressing PEDF demonstrated a reduction of laser-induced CNV of 33% when injected into the subretinal space, compared with 40% when injected into the vitreous.31 Because subretinal injections are more invasive, any increase in potency may be outweighed by the risks associated with the injection, such as retinal detachment.
Though intravitreal injections are part of routine care for many patients with ocular neovascularization, they do carry risks for potentially blinding complications.16 In contrast to subretinal and intravitreal injections, periocular injections are less invasive; however, they require the movement of material through the sclera and choroid in order to reach the inner structures of the eye. Periocular delivery involves the injection of material between the conjunctiva and the external surface of the sclera and avoids many complications of intraocular injections as the sclera itself is not injured.130 Experiments using Green Fluorescent Protein (GFP) protein diffusion showed levels in the retina and RPE at levels comparable to intravitreal experiments, although the authors note that physiologically relevant proteins, like PEDF or sFlt-1, are larger than GFP and may have slower kinetics.130 Naked siRNA against VEGFR-1 delivered via periocular injection diffused throughout the retina and inhibited neovascularization.72 Subsequent studies examining gene delivery using adenovirus showed localization of LacZ along the sclera and outside surface of the orbit,131 with diffusion of PEDF as far as the choroid and RPE.131 In fact, periocular delivery of adenovirus expressing PEDF showed a significant increase in PEDF levels in the RPE and choroid compared to the same virus injected into the vitreous, although no PEDF was detected in the retina.132 Periocular injection has proved effective in the rescue of CNV with an adenovirus expressing either PEDF131,132 or sFlt-1,132 as well as in the rescue of corneal angiogenesis using AAV expressing angiostatin.133 Studies using a pig model of laser-induced CNV, in which scleral thickness and orbit size are more similar to humans, demonstrated that even under these conditions periocular injection of a PEDF expressing adenovirus results in a 77% reduction in CNV.134 However, since PEDF was shown only to penetrate the RPE and choroid but not the retina, predictably periocular injection was unable to rescue an ROP model of RNV.131,132 Thus, periocular gene delivery may not be a viable option for the treatment of retinal angiogenesis.
Intravenous administration is also an attractive delivery method for gene therapy since it avoids complications of intraocular delivery. However, systemic delivery of antiangiogenic molecules has the potential for side effects that may make them poorly tolerated. While injection of secreted gene therapy agents has shown efficacy in inhibiting ocular neovascularization, it has also shown a concomitant increase in serum levels of the protein.27,30 Recent efforts have sought to target gene delivery specifically to the neovascular site, thus decreasing systemic exposure to the angiostatic protein. Evidence using functionalized nanoparticles85 and pseudotyped AAV that target the vasculature indicate that intravenous injection may be a safe form of delivery. While restricting the site of exposure can increase transduction of specific cell types, transgenes can be further controlled by the use of promoters and enhancers that restrict the site of expression.
REGULATION OF GENE EXPRESSION
While gene therapy offers the potential for long-term drug delivery, sustained and unregulated expression of angiostatic factors could result in deleterious side effects to nearby tissue. VEGF mediates important housekeeping functions in the mature retina and acts as a survival factor for the vascular endothelium. Regulation of gene expression can be accomplished through a number of molecular mechanisms, including vector targeting of specific tissues, use of inducible promoters, and/or tissue response elements.
Targeting dividing cells may be a way to restrict tissue transfection to sites of neovascularization. In the laser-induced CNV model, adenovirus has been shown to preferentially infect the dividing RPE cells at the site of injury.135 Integrins, such as αvβ3, have been shown to be upregulated in neovascular blood vessels in proliferative diabetic retinopathy and AMD patients136 and transferrin has been shown to be highly expressed in the retinas of AMD patients.137 Capitalization of these properties by functionalization of nanospheres, with either a peptide that binds the αvβ3 integrin or transferrin, has been shown to selectively increase transduction in neovascular ocular tissue.85 Clinically, infants suffering from ROP have an initial state of hypoproliferation and there is concern that blanket antiangiogenic treatment could also block the healthy development of blood vessels. Developmental markers that distinguish between pathologic angiogenesis and normal blood vessel vasculogenesis may provide potential targets for gene therapy vectors.138
Tissue-specific promoters are another mechanism by which to target gene expression. Commonly used promoters such as the cytomegalovirus (CMV) enhancer-promoter and CMV enhancer-chicken beta-actin (CBA) promoter both drive expression in multiple cell types. Additionally, it should be noted that, while both the CMV and CBA promoters are considered ubiquitous, studies using Ad5 have shown that changing the transgene promoter from CMV to CBA dramatically increased photoreceptor expression.101 Changing the promoter can ensure that gene expression is limited to a select cell population; however, large regulatory elements can exceed the cloning capacity of many viruses, especially AAV. Recently, smaller photoreceptor-specific promoters have been described that may circumvent the need for large regulatory elements, including a 470 bp139 and a 235 bp promoter,140 that limit expression to photoreceptors.
Inducible promoters allow for the selective activation of gene expression, often via an antibiotic response element. A few of these promoters have been described and have shown efficacy in the eye, such as the tetracycline inducible promoter expressed from either an adenovirus109,141 or AAV142 vector and the rapamycin inducible promoter expressed from an AAV vector.143 The CMV promoter, while expressing a high level of transgene in most tissues, has been shown to have transient expression, with either little or no expression within a few weeks.144 In a recent study, PEDF expression by the CMV promoter returned to baseline 90 days after adenoviral vector injection into the vitreous.144 However, a systemic injection of all-trans retinoic acid was able to restimulate transgene expression and the level of reactivation was high enough to inhibit laser-induced CNV.144 While the use of inducible promoters allows for tighter regulation of transgene expression, there are a number of drawbacks including the need for regular and more sensitive clinical observation for early detection of neovascularization, potentially inefficient drug penetration across the blood-retinal barrier, and the side effects of long-term antibiotic dosing.
An alternative to small molecule induced promoter regulation is the use of tissue response element promoters, which allow for transgene activation in response to the local tissue environment. Hypoxia is a driving stimulus for many antiangiogenic molecules and is a known pathogenic factor in ROP and diabetic retinopathy. Hypoxia response elements (HREs) tightly regulate gene expression to respond only to local tissue hypoxia. HREs regulate a number of different genes by binding hypoxia inducible factor 1α (HIF-1α), including VEGF.145 The use of HRE sequences to regulate transgene expression has proven effective in other models of disease such as myocardial ischemia.146 In experimental models of both RNV and CNV, HRE-mediated GFP expression from an AAV vector occurred only in sites of neovascularization during the early stages of angiogenesis.147 Regulation using HRE and other tissue response regulatory sequences offers a promising tool to overcome potential complications of constitutive expression of antiangiogenic factors.
ANIMAL MODELS AND RESCUE
A number of different animal models have been used to study ocular angiogenesis (for a complete review see Ref. 148). To date, a wide variety of vectors and transgenes have been shown to prevent angiogenesis using animal models of ocular neovascularization (Table 26.4). Gene therapy has shown efficacy in treating models of neovascularization in various tissues of the eye, including laser-induced disruption of Bruch membrane, hypoxia-induced RNV model of ROP, experimentally induced corneal angiogenesis, as well as VEGF overexpression (Table 26.5).
Table 26.4.
Gene therapy vectors used to rescue animal models of neovascularization
Vector | Site | Cells | Molecule | Model | Result | References |
---|---|---|---|---|---|---|
BIV | SR | RPE | Endostatin | VEGF-TG | Decreased RNV and permeability | (94) |
HIV | IV | Low GC, INL, ONL | Angiostatin | ROP | 90% decrease in RNV | (153) |
Ad5 | IV | CE, IPE | sFlt-1 | ROP | 56% decrease in RNV | (49) |
Ad5 (second generation) | IV | GC, INL | sFlt-1 | ROP | 97.5% decrease in RNV | (159) |
Ad5 | IM | sFlt-1(fused to Ig Fc portion) | Laser | Decreased CNV and inflammation | (150) | |
Ad5 (second generation) | IV | CE, trabecular meshwork | sFlt-1 | Corneal NV | 80% decrease in corneal NV | (160) |
Hd-Ad5 | IV | MC | sFlt-1 (DOX) | ROP | 60% decrease in RNV (dox dependent) | (109) |
Ad5 (second generation) | PO | Extra-ocular tissue | PEDF | Laser | 80% decreased CNV area | (131) |
PO | ROP | No reduction in RNV | ||||
Ad/F35 RGD | IV | ND | PEDF | Laser | 80% decrease in CNV | (102) |
Ad5 (second generation) | PO | Extraocular tissue | sFlt-1 | Laser | 82% decrease in CNV, reduced permeability | (132) |
PO | ROP | No reduction in RNV | ||||
IV | ND | Laser | 52% decrease in CNV | |||
Ad5 (second generation) | IV | ND | PEDF | Laser | 33% decrease in CNV | (28) |
IV | ROP | 50% decrease in RNV | ||||
IV | VEGF-TG | 80% decrease in RNV | ||||
SR | ND | Laser | 70% decrease in CNV | |||
Ad5 (second generation) | IV | ND | PEDF | Laser | 33% CNV regression | (152) |
SR | ND | Laser | 50% CNV regression | |||
SR | VEGF-TG | 80% RNV regression | ||||
Ad5 (second generation) | IV | CE | Anti-VEGF RNA | Corneal NV | Decreased VEGF levels, 86% decrease in NV | (69) |
Ad5 (second generation) | SR | RPE | Anti-VEGF shRNA | Ad5-VEGF | 84% CNV reduction | (75) |
Hd-Ad5 | SR | RPE | Endostatin (TAM) | VEGF-TG | Decreased RNV and permeability | (94) |
Ad5 (second generation) | TV | liver | Endostatin | Laser | No detectable CMV | (30) |
Ad5 | IM | |||||
IM | ExTek | Laser | 45% decrease in CNV | (27) | ||
ROP | 47% decrease in RNV | |||||
Ad5 | SR | RPE, ONL | VHL | BRVO | Decreased RNV | (84) |
AAV2 | SR | RPE, ONL | sFlt-1 | Laser | 19% reduction in CNV | (129) |
IV | CE, IPE | Corneal NV | 36% reduction in corneal NV | |||
AAV2 (P2 injection) | IV | GC, INL | sFlt-1 | ROP | 52% reduction in RNV | (49) |
AAV2 | SR | RPE, ONL | sFlt-1 | VEGF-TG | 85% decrease RNV, ERG rescue, decreased permeability | (151) |
SR | Laser (monkey) | No detectable CNV | ||||
AAV2 | SR | RPE, ONL | PEDF | Laser | 33% decrease CNV area | (31) |
IV | GC | 40% decrease CNV area | ||||
AAV2/1 | SR | RPE | PEDF | ROP | 85% decrease in RNV | (26) |
SR | Endostatin | 82% decrease in RNV | ||||
SR | TIMP-3 | 75% decrease in RNV | ||||
AAV2 | PO | Cornea | Endostatin | Corneal NV | No corneal NV | (161) |
AAV2 | SR | ND | PEDF | ROP | 74% decrease NV cells | (63) |
Angiostatin | 78% decrease NV cells | |||||
AAV2 | SR | ND | Angiostatin | Laser | 40% reduction CNV area, rescue at 150 days postinjection | (29) |
AAV2 | PO | Extra-ocular tissue | Angiostatin | Corneal NV | Decreased corneal NV | (133) |
AAV2 | IV | GC, INL | ZFP-TF | Laser | Decreased CNV but not permeability, effect seen out to 3 months | (76) |
SR | RPE | Activating PEDF | ||||
PGLA nanoparticles | TV | RPE | Anti-VEGF intraceptor | Laser | 50%–75% reduction in CNV | (85) |
FuGENE 6 | PO | Cornea | GABP | Corneal NV | Reduced VEGF and Robo4 expression, decreased corneal NV | (77) |
Lipofectamine 2000 | IV | Retina | siRNA against HIF-1 and VEGF | ROP | Decreased RNV | (73) |
Direct injection | PO | GC, some INL, ONL | siRNA against VEGFR-1 | Laser | 45% reduction in NV | (72) |
IV | GC, some INL, ONL | 66% reduction in NV |
Ad5, adenovirus; Ad5-VEGF, adenovirus expressing VEGF; BIV, bovine immunodeficiency virus; BRVO, multiple branch retinal vein occlusion; CE, corneal endothelium; CNV, choroidal neovascularization; DOX, doxycycline inducible; ExTek, soluble Ang2 receptor; GC, ganglion cells; Hd-Ad, helper-dependent adenovirus; Ig, immunoglobulin; IM, intramuscular; INL, inner nuclear layer (bipolar cell nuclei); IPE, iris pigment epithelium; IV, intravitreal; ND, not determined; NV, neovascular; ONL, outer nuclear layer (photoreceptor nuclei); PO, periocular; RNV, retinal neovascularization; ROP, retinopathy of prematurity hypoxic model; RPE, retinal pigment epithelium; SR, subretinal; TAM, tamoxifin inducible; TV, tail vein; VEGF-TG, VEGF transgenic; ZFP-TF, zinc-finger protein transcription factor.
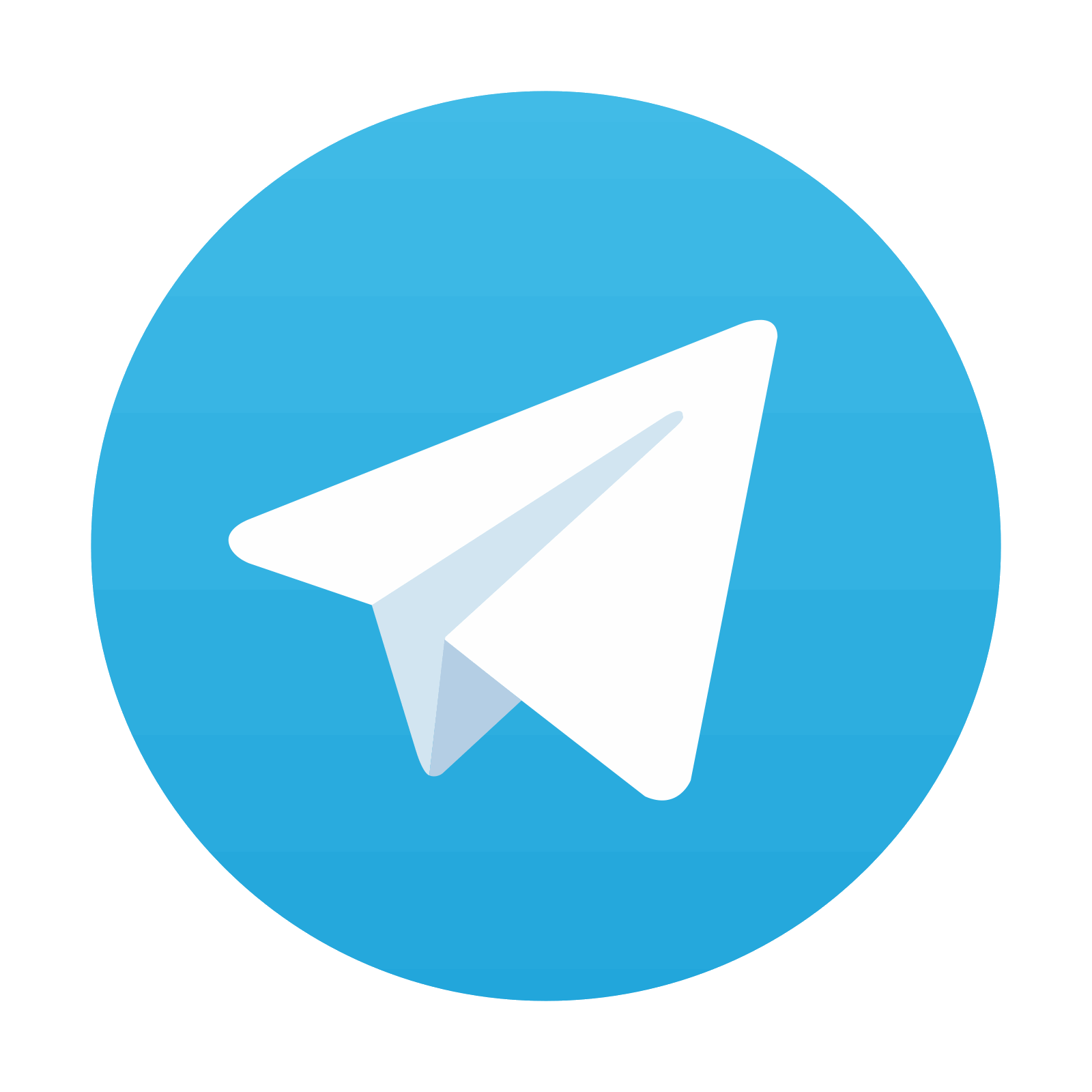
Stay updated, free articles. Join our Telegram channel
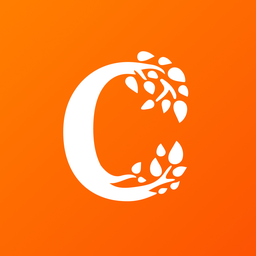
Full access? Get Clinical Tree
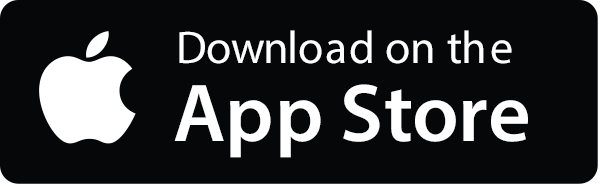
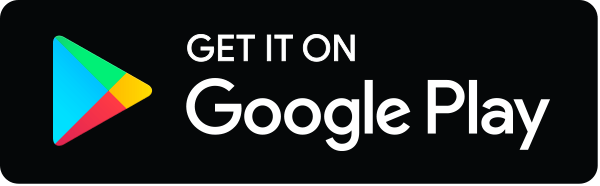