in the Management of Age-Related Macular
Degeneration and Macular Edema
Pearse A. Keane • Srinivas R. Sadda
INTRODUCTION TO OPTICAL COHERENCE TOMOGRAPHY
Optical coherence tomography (OCT), first described by Huang et al.1 in 1991, allows high-resolution cross-sectional (tomographic) images of the neurosensory retina to be obtained in a noninvasive manner. Commercially available OCT systems are now capable of obtaining retinal images with an axial resolution of approximately 5 to 8 μm, and a transverse resolution of approximately 15 to 20 μm.2 As a result, OCT has been characterized as in vivo “clinical biopsy” and has transformed the diagnosis and management of vitreoretinal disorders.3
BASIC PRINCIPLES
OCT works by measuring the properties of light waves reflected from, and scattered by, tissue (analogous to ultrasonography).4 The use of light waves in OCT results in images with much greater resolution than ultrasonography as the wavelength of light is many times less than that of sound. However, the use of light instead of sound is challenging as the speed of light exceeds the speed of sound by a factor of 150,000 times, making direct measurements of optical “echoes” impossible over small distances. OCT instruments employ a number of the distinctive physical properties of light waves to overcome this technological hurdle, in particular the principle of interference.
Interference is a phenomenon that occurs when two light waves meet each other and are superimposed. The resulting waveform depends on the wavelength, amplitude, and relative phase of the two waves. Interferometry uses the principle of interference to deduce the original state of waves by analyzing the result of their combination.4 In interferometers, a single beam of light is split into two identical beams—each beam travels a separate path before being recombined together at a single detector. The combination of light reflected from the tissue of interest, and light reflected from a reference mirror, produces characteristic patterns of interference that are diagnostic of the mismatch between the reflected waves. In the earliest OCT systems, these interference patterns were assessed as a function of time—“time domain” OCT.
TIME DOMAIN OCT
In 1993, the first time domain OCT device capable of in vivo use in humans was developed.5 This technology was acquired by Humphrey Instruments (a division of Carl Zeiss Meditec, Dublin, California), who subsequently developed the first commercial devices—OCT1 and OCT2. Improvements in the technology led to the development of OCT3—termed StratusOCT, currently the most commonly used OCT system worldwide. Although time domain OCT systems are capable of generating high-resolution images (8–10 μm for StratusOCT), they require the use of a mobile reference mirror for the assessment of interference patterns—a requirement that limits their image acquisition speed (400 A-scans per second for StratusOCT).4 This is a major restriction for clinical practice as only sparse coverage of the macular area is possible when acquiring any given image set.6 Fortunately, this technological hurdle has been overcome in recent years with the introduction of “spectral domain” OCT—a technology that removes the requirement for a mobile reference mirror by the assessment of interference patterns as a function of frequency rather than of time.7
SPECTRAL DOMAIN OCT
Spectral domain OCT systems use spectral interferometry and a mathematical function (Fourier transformation) to assess interference patterns as a function of frequency.8,9 Thus, light scattered from different depths within the tissue can be measured simultaneously, and images can be acquired 50 to 100 times more quickly than in time domain systems (typically over 20,000 A-scans per second).
The first in vivo imaging of the human retina using spectral domain OCT was reported in 2002.10 More recently, a number of commercial OCT systems, which incorporate spectral domain technology, have been released. These devices include Cirrus HD-OCT (Carl Zeiss Meditec, Dublin, CA), 3D OCT-1000 (Topcon Medical Systems, Japan), Spectralis OCT (Heidelberg Engineering, Germany), RTVue-100 (Optovue Corporation, Fremont, CA), Spectral OCT SLO (Opko Instrumentation/OTI, Canada), SOCT Copernicus HR (Reichert Ophthalmic Instruments, New York, NY), and 3D SDOCT (Bioptigen, NC). In addition to their increased speed and sensitivity, many of these instruments offer incremental improvements in axial resolution through the use of improved light sources.
Each of the recently introduced commercial spectral domain OCT systems is capable of acquiring sizable image sets over short time periods. As a result, new methods of utilizing these image sets have evolved, significantly enhancing the evaluation of vitreoretinal disorders.
RETINAL IMAGING WITH SPECTRAL DOMAIN TECHNOLOGY
Raster Scanning
The high speed of spectral domain OCT facilitates significantly greater sampling of the macular area via the use of raster scanning protocols.11–13 Raster scans consist of a rectangular pattern of horizontal line scans that run in parallel across the macula (e.g., 128 B-scans, with each B-scan consisting of 512 A-scans). The greater retinal sampling density of spectral domain raster scans may facilitate early detection of morphologic changes in disease states, as well as allowing these changes to be more accurately followed over time.
When dense raster scanning is performed, spectral domain OCT systems have the ability to perform volumetric rendering of the OCT images (rendering is the processing of OCT images to make them appear solid and three dimensional [3D]). Volumetric rendering allows enhanced visualization of the retina, which may take place from any direction, and which may be useful in disorders such as epiretinal membrane (ERM), or vitreomacular traction.14
OCT Fundus Images
Another important feature of spectral domain OCT is the ability to generate “OCT fundus images” that mimic the images obtained from standard fundus photography.12,13 These images are generated from the raster scan OCT data by summing the intensity of pixels in the axial direction, resulting in a pixel brightness value for each axial scan position. OCT fundus images show a direct view of the macula in which the retinal vascular arcades may be clearly visible and spatially consistent with the vasculature on color photographic, or angiographic, images. OCT fundus images are useful in the clinical setting as they can facilitate registration of any point on an OCT image with a corresponding point on the retinal surface. OCT fundus images also permit the acquisition of images in the same location over time, although this is not always possible in the setting of severe disease (where the vascular pattern and other landmarks may be less apparent) or in patients with poor fixation.
Other Features
Although it is possible to generate large 3D data stacks with spectral domain OCT, motion artifacts (e.g., vertical microsaccades) remain a problem when very high sampling density is required. Many spectral domain OCT systems address this problem by sacrificing sampling density along one axis of the raster scan; however, some commercially available OCT instruments (e.g., Spectralis, Heidelberg Engineering, Germany) also provide real-time eye motion tracking with the potential for longer acquisition times and higher density data sets.2
The rapid scanning of spectral domain OCT also allows multiple B-scans to be averaged, which reduces speckle noise and allows detailed visualization of fine structures—in many cases B-scan averaging results in images that rival or surpass prototype “ultrahigh resolution” systems.15
IMAGE INTERPRETATION IN NORMAL EYES
The high axial resolution offered by OCT is particularly well suited to assessment of the multilayered retinal structure (Fig. 20.1).4 On OCT false-color B-scans, highly reflective tissue is reddish-white in color, while hyporeflective tissue is blue-black in color.16 In most scans, the first hyperreflective layer detected is the internal limiting membrane (ILM) at the vitreoretinal interface. In a subset of the population, the posterior hyaloid may be seen as a thin hyperreflective layer above the ILM. Within the retina, the ganglion cell layer and both the inner and outer plexiform layers are seen as hyperreflective layers while the inner and outer nuclear layers are hyporeflective.
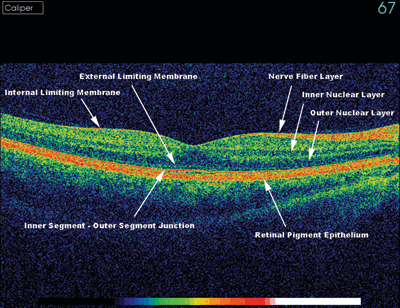
FIGURE 20.1. Optical coherence tomography B-scan produced using the 3D-OCT 1000 system (Topcon Medical Systems, Japan). The high axial resolution offered by OCT is well suited to providing information regarding the multilayered structure of the retina.
Correlation of OCT images with the microstructure of the outer retina is less well defined.17 The first continuous hyperreflective line typically seen in the outer retina corresponds to the junction of the inner and outer segments of the photoreceptors (IS-OS junction). A faint, less continuous hyperreflective line may be present above this line and is thought to represent the external limiting membrane (ELM). Beneath the IS-OS junction, the interdigitations of the photoreceptor outer segments and apical microvilli of the retinal pigment epithelium (RPE) may be visible with high-resolution OCT systems. Finally, a wide, hyperreflective line corresponding to the RPE-Bruch membrane-choriocapillaris complex lies at the outermost extent of these tissue layers. Using spectral domain OCT, larger choroidal vessels, the suprachoroidal space, and the choroidal-scleral junction may also be seen in good quality images.18,19
Quantitative Image Analysis
The high axial resolution offered by OCT is also well suited to the objective, accurate measurement of retinal thickness. StratusOCT uses image processing techniques to automatically detect the inner and outer retinal boundaries on OCT B-scans (segmentation) and thus provide measurements of retinal thickness.4 Using these techniques, it is possible to measure retinal thickness at multiple locations and to construct retinal thickness maps corresponding to the Early Treatment of Diabetic Retinopathy Study (ETDRS) subfields. Caution is required in the use of this information as errors in retinal segmentation are known to occur, and these errors are often severe in macular disorders such as neovascular age-related macular degeneration (AMD).20–29
As with StratusOCT, spectral domain OCT systems include image analysis software that provides measurements of retinal thickness. The speed and image resolution advantages of these systems may facilitate improved accuracy of retinal segmentation; however, further improvement in image processing algorithms may be required before segmentation errors can be eliminated entirely, particularly for disorders with complex morphology.30,31
AGE-RELATED MACULAR DEGENERATION
AMD is the leading cause of irreversible visual loss in people aged 50 years or older in the developed world.32 The clinical hallmark of AMD is the deposition of acellular, polymorphous material, termed drusen, between the RPE and Bruch membrane.33 In early AMD, drusen are often accompanied by focal retinal pigmentary abnormalities. As AMD progresses, alterations in the RPE often accumulate, resulting in the loss of large areas of RPE and outer retina, a phenomenon termed geographic atrophy (GA). In some patients with AMD, the abnormalities in the outer retina, Bruch membrane, and choroid may also result in the development of choroidal neovascularization (CNV), the characteristic feature of neovascular AMD.
In neovascular AMD, abnormal blood vessels develop from the choroidal circulation, pass anteriorly through breaks in Bruch membrane, and then proliferate in the sub-RPE or subretinal space.34 CNV lesions may thus result in pigment epithelium detachment (PED), fluid exudation, lipid deposition, subretinal hemorrhage, and ultimately fibrotic scar formation with irreversible visual loss. For many years, fluorescein angiography (FA) was the cornerstone of neovascular AMD treatment. However, with the recent introduction of antiangiogenic agents, e.g., ranibizumab (Lucentis; Genentech, South San Francisco, CA) and the need for frequent monitoring of patients receiving these treatments, OCT has emerged as an essential tool in the follow-up of patients with AMD.35,36
FEATURES OF AGE-RELATED MACULAR DEGENERATION ON OCT
Pigment Epithelium Detachment
In neovascular AMD, growth of CNV in the sub-RPE space produces an elevated lesion that is often visible on clinical examination and is termed a fibrovascular PED.34,37 Lesion growth is often accompanied by leakage of serous fluid, or frank hemorrhage, leading to the formation of serous or hemorrhagic PEDs. On OCT, PEDs appear as broad elevations of the RPE band relative to Bruch membrane (Fig. 20.2A).38 The boundaries of a PED may be either gently sloped (as with some fibrovascular PEDs) or steeply sloped (as with many serous PEDs), although it is often not possible to distinguish between PED subtypes with confidence using OCT. In serous PEDs, Bruch membrane is often visible as a thin hyperreflective line at the outer aspect of the PED; Bruch membrane is less commonly seen in hemorrhagic PEDs, where there is often dramatic posterior shadowing below the RPE.
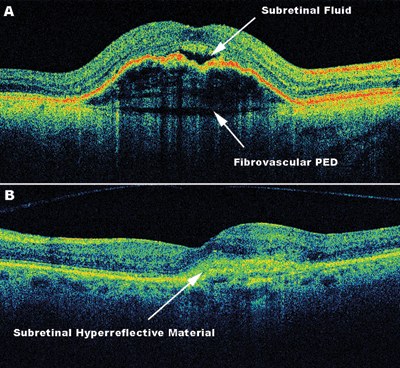
FIGURE 20.2. Optical coherence tomography B-scans (Cirrus HD- OCT, Carl Zeiss Meditec, Dublin, CA) demonstrating fibrovascular pigment epithelial detachment (PED) (A) and subretinal hyperreflective material (B).
The detailed structural characteristics and precise mechanisms of PED formation have not been completely resolved—in part due to the relative inability of conventional OCT devices to visualize the areas underneath the highly reflective RPE. Recent studies have utilized enhanced spectral domain OCT imaging to aid visualization of the sub-RPE space.18,19,39 Using this method, many fibrovascular PEDs appear to be filled with solid layers of material of medium reflectivity, separated by hyporeflective clefts—a finding consistent with previous histopathologic reports.40,41 In many serous PEDs, collections of solid material—the apparent fibrovascular proliferation—can be seen adherent to the outer surface of the RPE, and associated with an underlying hyporeflective space—the serous fluid compartment. Following treatment with antiangiogenic agents, these supposed areas of sub-RPE CNV can be seen to contract and separate from the RPE, with flattening of the PED, resulting in RPE tear formation in a minority of cases (see below).39
Subretinal Hyperreflective Material
In many cases of neovascular AMD, the abnormal blood vessels may pass directly into the subretinal space after their initial penetration of Bruch membrane.34 In these initial growth phases, the CNV membrane is often highly vascular and appears on OCT as an amorphous lesion of medium- to high-reflectivity above the RPE (Fig. 20.2B).42,43 As the CNV lesion becomes less active over time, the vascular component typically regresses, while the fibrous component increases, resulting in disciform scar formation that appears on OCT as a well-demarcated highly hyperreflective lesion. Scar formation may be associated with loss of the overlying photoreceptor layer and irreversible reduction in visual acuity—this may often be seen on OCT as disruption of the IS-OS junction.44,45 Hemorrhage, lipid, or thick fibrin may also appear as hyperreflective material in the subretinal space but should not be mistaken for the fibrovascular lesion.
Subretinal Fluid
As the choroidal neovascular membrane grows, it is often accompanied by profuse leakage from its immature blood vessels. Consequently, pockets of fluid commonly accumulate between the neurosensory retina and the RPE; and these areas may be seen on OCT as hyporeflective spaces (Fig. 20.2A).42 CNV lesions growing in the subretinal space are typically associated with the greatest volume of subretinal fluid; however, sub-RPE lesions may also result in subretinal fluid when there is sufficient dysfunction of the outer blood-retinal barrier.46 When fluid exudation is serous in nature, subretinal fluid pockets are seen on OCT as homogenous hyporeflective spaces; when the exudate contains fibrin or red blood cells, the area of subretinal fluid may by sparsely hyperreflective. Profuse fibrinous exudation in neovascular AMD, seen after PDT or in particularly active classic CNV lesions, may result in the formation of distinct subretinal fluid compartments separated by fibrinous membranes—these compartments may sometimes be seen on FA as areas of loculated fluid.47 Spectral domain OCT allows enhanced visualization of the subretinal space, and assessment of the optical density of subretinal fluid compartments may have value for the differentiation of macular disorders associated with subretinal accumulation.48
Intraretinal Fluid
Disruption of the ELM-photoreceptor complex in the outer retina, by the active CNV membrane, may result in the accumulation of fluid in the neurosensory retina.49 Initially, this fluid collection manifests as diffuse thickening of the outer nuclear layer.50 Severe outer nuclear layer swelling may sometimes be mistaken for subretinal fluid—the distinction can usually be made by the presence of an intact IS-OS junction at the outer aspect of outer nuclear layer thickening. With more severe fluid exudation, cystoid spaces may form and can be seen on OCT as round or oval hyporeflective areas (Fig. 20.3).50,51 Larger cystoid spaces often contain tissue septae and may involve all layers of the retina. Treatment with antiangiogenic agents often leads to cessation of CNV leakage with consequent resolution of these cystoid spaces. In severe cases, however, cystoid spaces may persist even in the absence of leakage, and these areas have been termed “cystoid macular degeneration.”52
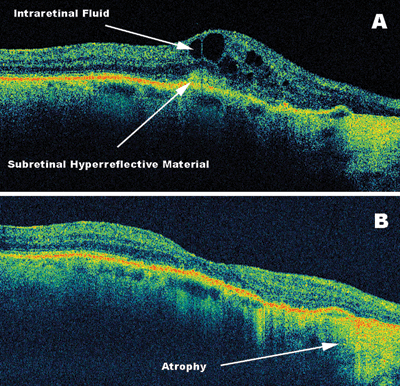
FIGURE 20.3. Optical coherence tomography B-scans (Cirrus HD-OCT, Carl Zeiss Meditec, Dublin, CA), demonstrating resolution of intraretinal fluid following treatment with ranibizumab (Lucentis, Genentech, South San Francisco, CA), in a patient with neovascular AMD. Pretreatment (A), posttreatment.
Drusen and Retinal Pigment Abnormalities
Drusen may sometimes be difficult to assess on time domain OCT images, where undulation of the RPE baseline due to motion artifact may mimic the appearance of drusen.53–55 On spectral domain OCT, small and intermediate size drusen may be more clearly seen as discrete areas of RPE elevation with variable reflectivity, reflecting the variable composition of the underlying material (Fig. 20.4A).56,57 In large drusen, or drusenoid PED, greater elevation of the RPE may be seen, often dome shaped, with a hyporeflective or medium-reflective material separating the RPE from the underlying Bruch membrane.54 Disruption of the IS-OS junction and ELM, as well as significant thinning of the outer nuclear layer, may often be seen overlying drusen.57 In patients with extensive drusen, apparent thickening of the photoreceptor outer segments may sometimes be seen—the accumulation of material in this area may represent incomplete phagocytosis of the outer segment material.58
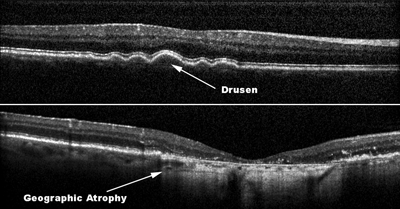
FIGURE 20.4. Optical coherence tomography B-scans (Spectralis, Heidelberg Engineering, Germany) demonstrating drusen (A) and geographic atrophy (B).
Drusen are often accompanied by RPE abnormalities that can be visualized on OCT.57 In particular, pigment migration and clumping may be seen as discrete areas of hyperreflectivity in the outer nuclear layer with underlying shadowing. Focal areas of RPE loss may result in increased reflectivity of the underlying choroidal vessels. A recent study has also shown that drusen may be associated with a diffuse hyperreflective haze at the inner boundary of the outer nuclear layer, although the origin and significance of this finding remain uncertain.59
Geographic Atrophy
In GA, confluent areas of RPE atrophy are accompanied by loss of the overlying photoreceptors.60 On OCT, GA appears as areas of sharply demarcated choroidal hyperreflectivity due to loss of the overlying RPE (Fig. 20.4B).61 GA is also accompanied by thinning of the overlying neurosensory retina that can be seen on spectral domain OCT as absence of the outer nuclear layer, ELM, and IS-OS junctions (these layers may be seen to taper off in the junctional zone surrounding GA).58 Islands of preserved outer retina may sometimes be identified in areas of GA, as can regressing drusenoid material, seen as hyperreflective plaques at the level of the RPE.58 Preliminary OCT evidence also suggests that mild retinal swelling in areas of foveal sparing may represent a preapoptotic stage of neuronal cellular elements indicative of imminent atrophy.62
The OCT fundus images provided by spectral domain OCT devices show an increased total signal in areas where GA is apparent on clinical examination.63 As a result, it is possible to manually quantify areas of GA by registering these images to fundus photographs and delineating the GA boundaries with imaging software. This approach may allow more accurate and reproducible measurements of GA progression in future clinical trials of this disorder.
Abnormalities of the Vitreomacular Interface
Retinal imaging with OCT allows detailed evaluation of the structural features of the vitreomacular interface. On OCT, vitreomacular traction may be seen when a thickened, taut, posterior hyaloid causes deformation of the inner retinal surface, which may often be accompanied by cystoid macular edema (CME).64 ERMs are often seen on OCT as hyperreflective bands anterior to the inner retinal surface, with distortion of the underlying anatomy (Fig. 20.5).65 In recent years, it has been suggested that abnormalities of this interface may play a role in CNV pathogenesis.66 A number of studies, employing both time domain and spectral domain OCT, have reported a higher prevalence of vitreomacular adhesion in patients with neovascular AMD.67–70 Further study is required to confirm this association and to determine whether it has any prognostic or patient management implications.
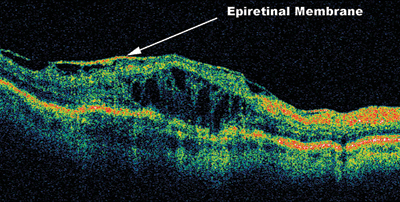
FIGURE 20.5. Evidence of dense ERM formation in a patient receiving treatment for neovascular AMD (Stratus OCT, Carl Zeiss Meditec, Dublin, CA).
CLINICAL APPLICATIONS OF OCT IN AGE-RELATED MACULAR DEGENERATION
Diagnosis
In elderly patients complaining of acute or subacute unilateral visual loss, the combination of biomicroscopic (e.g., drusen, macular hemorrhage, subretinal fibrosis) and tomographic (e.g., PED, subretinal hyperreflective material, subretinal fluid) findings often allows a diagnosis of neovascular AMD to be made with confidence.71 This is particularly the case with the advent of spectral domain OCT, where areas of focal macular pathology are less likely to be missed with raster scanning of the macula. However, clinical studies have not yet adequately addressed this issue, and FA is still regarded by most retinal specialists as mandatory for the initial diagnosis of neovascular AMD.35 Aside from its emerging diagnostic role, OCT allows assessment of disease activity and, in this context, has become essential in guiding the treatment of patients with neovascular AMD.
Role in Retreatment Protocols
In 2006, the MARINA and ANCHOR phase III clinical trials demonstrated that treatment with ranibizumab was capable of improving visual acuity in many patients with neovascular AMD.72,73 In parallel with this, use of OCT had become widespread among retinal specialists for the management of neovascular AMD. Consequently, OCT measurements were soon adopted as anatomic outcome parameters, and as a means of guiding retreatment, in clinical trials. In the PrONTO study, an OCT-guided variable dosing regimen was assessed for the treatment of neovascular AMD with ranibizumab.74 In this study, ranibizumab re-treatment was performed, in part, based on OCT criteria: a loss of five letters of visual acuity in conjunction with fluid on OCT, an increase of OCT central retinal thickness of at least 100 μm, or the persistence of fluid on OCT 1 month after previous treatment. In the second year of this study, these criteria were amended so that any qualitative OCT changes suggestive of recurrent fluid in the macula (e.g., the appearance of retinal cysts or subretinal fluid or enlargement of a PED) were indications for re-treatment.75 The results of this study suggested that an OCT-guided re-treatment regimen could result in visual outcomes comparable to those from clinical trials with fixed monthly dosing. The efficacy of this approach is now being evaluated in large-scale clinical trials—in particular, the CATT (Comparison of Age-Related Macular Degeneration Treatments Trials) and the SUSTAIN (Study of Ranibizumab in Patients With Subfoveal Choroidal Neovascularization Secondary to Age-Related Macular Degeneration) trials.
Correlations with Visual Acuity
Many clinical trials have demonstrated substantial changes in retinal thickness in response to antiangiogenic therapies.76–78 However, many of these studies have failed to find a significant correlation between OCT-derived retinal thickness and visual acuity.77,79 This failure may be due, at least in part, to the frequent inaccuracy of retinal thickness measurements provided by OCT image analysis software.28 Manual segmentation of OCT images allows accurate measurement of retinal thickness, as well as allowing quantification of any morphologic space of interest in the retina (e.g., intraretinal cysts, subretinal hyperreflective material, subretinal fluid, or PED).80,81 Using this approach, modest correlations have been found between the volume of subretinal hyperreflective material, the thickness of the neurosensory retina, and visual acuity in patients newly diagnosed with neovascular AMD.46 Manual grading has also shown that regression of any anatomic improvement gained after treatment with ranibizumab is strongly associated with reduction in visual acuity.82 Manual segmentation has usually been performed using custom software, although OCT image analysis software from certain vendors has begun to offer this facility (e.g., Stratus OCT v5.0). Spectral domain OCT may allow more accurate quantification of retinal pathology, although manual grading of these images may not be feasible for large studies.48,84–85
Correlation with Fluorescein Angiography
CNV lesions in neovascular AMD are commonly described as “classic” or “occult”, depending on their leakage characteristics on FA.86 Classic CNV is believed to correspond to fibrovascular proliferation in the subretinal space, while occult CNV is believed to correspond to fibrovascular proliferation beneath the RPE.34,87 This classification may have clinical significance in terms of disease progression over time and impact on visual function. A number of studies have examined the relationship between OCT findings and FA in neovascular AMD.38,88,89 In these studies, classic lesions are associated with significantly greater volumes of subretinal hyperreflective material than occult lesions, while occult lesions are more commonly associated with PED. Studies of this manner are first steps toward development of hybrid OCT-FA classifications systems for neovascular AMD—the development of such systems may allow clinicians to identify those patients most likely to respond to any given therapeutic intervention and thus improve clinical outcomes.
OTHER FEATURES OF AGE-RELATED MACULAR DEGENERATION ON OCT
Retinal Angiomatous Proliferation
Although neovascular AMD is predominantly a disorder of the choroidal vasculature, the retinal circulation may also be involved in some patients, and retinochoroidal anastomoses may form in advanced disease.90 In 2001, Yannuzzi et al.91 suggested that the initial event in the development of retinochoroidal anastomosis was intraretinal neovascularization and coined the term retinal angiomatous proliferation (RAP). Conversely, Gass et al.92 suggested that the initial event in retinal involvement was the development of occult anastomosis at the site of a sub-RPE CNV lesion. A recent histopathologic study has provided evidence supportive of an intraretinal origin for the neovascular process in these patients.93 However, recent OCT studies suggest that the earliest change visible in these patients is the development of fibrovascular PED—a feature more consistent with the Gass theory of occult chorioretinal anastomosis.94,95 Thus, it has been suggested that retinal involvement in neovascular AMD may incorporate both sub-RPE and intraretinal origins (“the expanded spectrum of RAP”).95 On OCT, active RAP lesions have a characteristic appearance, typified by frank CME overlying a serous PED, and accompanied by subretinal fluid.96,97 In some cases, the retinochoroidal anastomosis may be seen on OCT as a discrete hyperreflective area extending through the retina.
Polypoidal Choroidal Vasculopathy
In 1990, Yannuzzi et al.98 suggested the term “idiopathic polypoidal choroidal vasculopathy” for a disorder characterized by multiple serosanguineous PEDs, commonly seen in black and Asian populations. This disorder was initially felt to be a distinct entity with its own risk factors and clinical course; however, in recent years, the disease spectrum has been greatly expanded, with many authorities now considering PCV to be an important form of neovascular AMD.99 On indocyanine green (ICG) angiography, PCV appears as a branching vascular network below the RPE that ends in reddish-orange polypoidal lesions and is often associated with serosanguineous PEDs.100 On Stratus OCT, and more recently with spectral domain OCT, the branching vascular network appears as a shallow elevation of the RPE, while the polypoidal lesions appear as sharper protuberances (Fig. 20.6).101–107 As the exudative complications of these lesions evolve, large serosanguineous PEDs develop adjacent to the polypoidal bulges, creating a tomographic notch. With continued exudation, these polypoidal lesions remain adherent to the RPE and are lifted away from Bruch membrane.107 ICG angiography is still considered essential for the diagnosis of PCV, although OCT is likely to play a greater role as the technology improves and the awareness of this disorder grows.
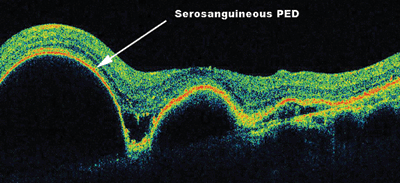
FIGURE 20.6. Optical coherence tomography B-scan (Cirrus HD-OCT, Carl Zeiss Meditec, Dublin, CA), demonstrating serosanguineous pigment epithelium detachments (PEDs) in a patient with polypoidal choroidal vasculopathy.
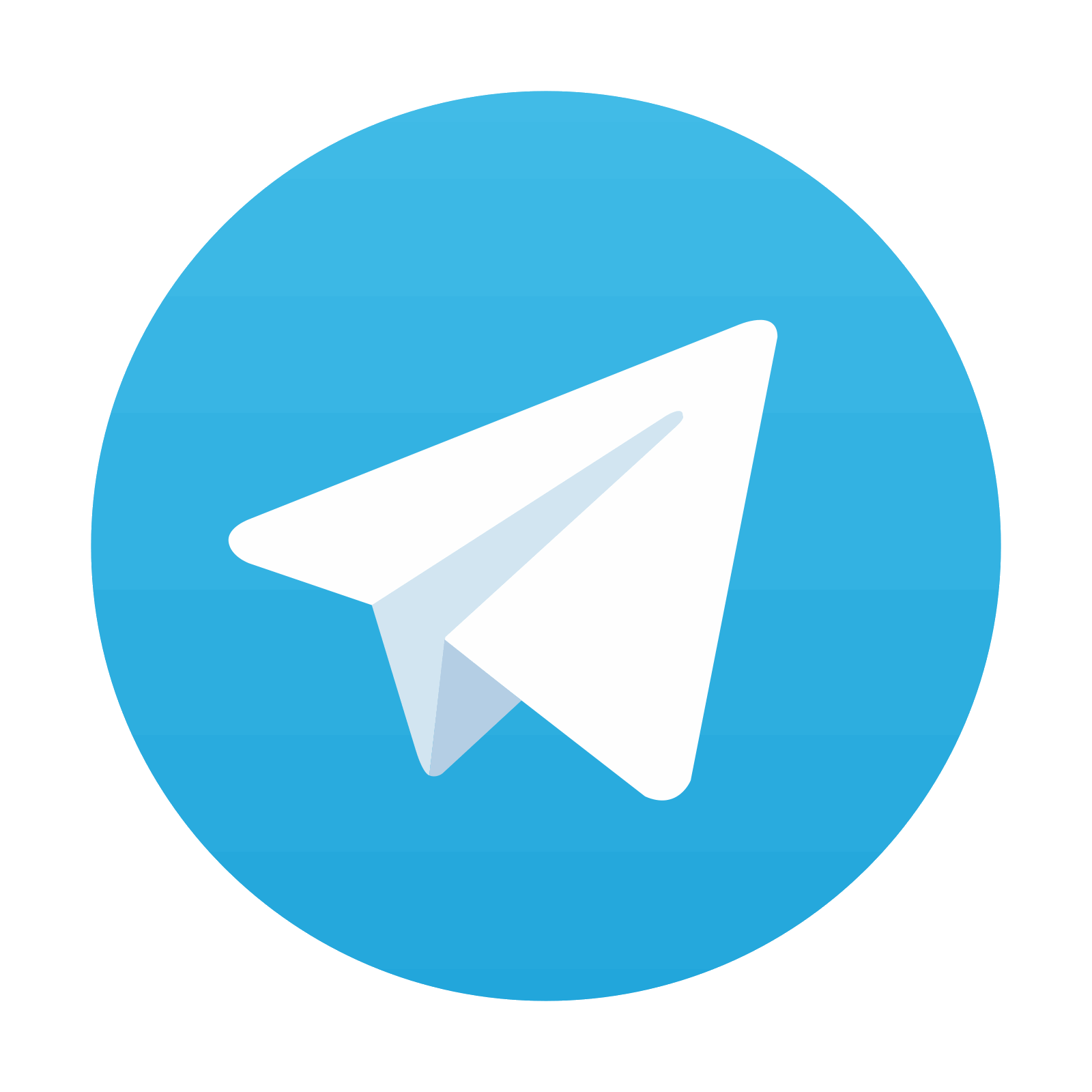
Stay updated, free articles. Join our Telegram channel
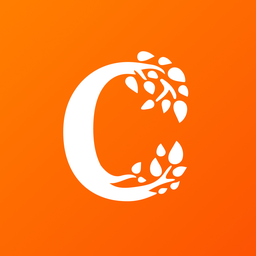
Full access? Get Clinical Tree
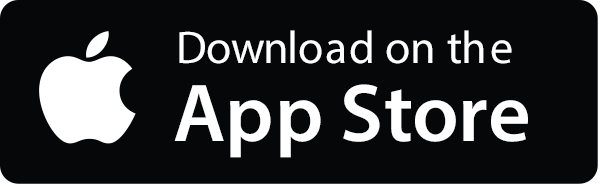
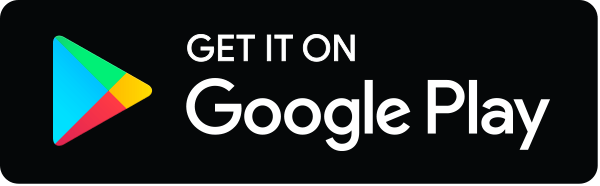