Abstract
Vitreous is an extended extracellular matrix that is important in various physiologic processes within the eye. The vitreous body undergoes changes with aging that at best cause floaters and at worst cause anomalous posterior vitreous detachment, often with vitreoschisis. The vitreoretinal interface is particularly important in the pathophysiology of various vitreomaculopathies. It is now understood that anomalous PVD plays a key role in a variety of retinal diseases, such as macular holes, macular pucker, and retinal detachment, as well as aggravating a number of comorbidities, such as diabetic retinopathy, retinal vein occlusions, and age-related macular degeneration. Enhanced understanding of the biochemistry, anatomy, and pathophysiology of vitreous will enable new therapeutic approaches such as is currently occurring with the replacement of surgery by pharmacologic vitreolysis.
Key words
vitreous, extracellular r matrix, collagen, hyaluronan, vitreoretinal interface, anomalous posterior vitreous detachment, vitreoschisis, vitreomaculopathies, vitreoretinal traction, peripheral retinal pathology
* W. Richard Green, MD was first author of this chapter for the first four editions of this book. His monumental contributions to this field are reflected in the histopathologic images and content of this chapter, which continues to teach long after his parting on July 5, 2010.
Biochemistry
Vitreous is 98% water and 2% structural proteins, extracellular matrix components, and miscellaneous compounds.
Collagen
Collagen is the major structural protein, consisting of heterotypic fibrils ( Fig. 23.1 ) similar to cartilage. Following vitrectomy, a type II procollagen is secreted in humans, but an actual vitreous body is not reformed, since reoperations reveal that the gel state of vitreous is not reestablished.

Type II collagen comprises 75% of the total collagen content in vitreous. There are considerable similarities between vitreous and cartilage collagens, perhaps explaining why inborn errors of type II collagen metabolism result in “arthro-ophthalmopathies,” manifesting similar phenotypic expression in joints and vitreous. Type IX collagen accounts for up to 15% of vitreous collagen, where it always contains a chondroitin sulfate glycosaminoglycan chain covalently linked to the α 2 (IX) chain at the NC3 domain, enabling the molecule to assume a proteoglycan form.
An important function of vitreous is maintaining transparency within the eye ( Fig. 23.2 ). Studies have shown that one of the minor collagens of vitreous is type XVIII, progenitor of endostatin, a potent inhibitor of angiogenesis.

Hyaluronan
Hyaluronan (HA) was first isolated from bovine vitreous in 1934. HA appears after birth, perhaps synthesized by hyalocytes, the ciliary body, and/or Müller cells. It is a large polyanion, which can influence the diffusion of drugs through vitreous. As a result of HA’s entanglement within the vitreous collagen fibril matrix, the mechanical force of HA’s extension and contraction can be transmitted to the retina, optic disc, and neovascular complexes, inducing untoward effects in conditions that have fluctuations in ionic balance and hydration, such as diabetes.
Chondroitin Sulfate
Most vitreous chondroitin sulfate is in the form of versican, believed to form complexes with HA as well as with microfibrillar proteins such as fibulin-1 and fibullin-2 and play a crucial role in maintaining the molecular morphology of vitreous. Mutations that alter the splicing of the central chondroitin sulfate-bearing domains of versican have been implicated in Wagner syndrome, a condition with excess vitreous liquefaction.
Noncollagenous Structural Proteins
Fibrillins
In Marfan syndrome, defects in the gene encoding fibrillin-1 ( FBN1 on chromosome 15q21) result in both ectopia lentis and vitreous liquefaction, an important cause of rhegmatogenous retinal detachment (RD) in these patients.
Opticin
A major noncollagenous protein of vitreous is opticin (formerly called vitrican). It is bound to the surface of the heterotypic collagen fibrils and prevents aggregation of adjacent collagen fibrils into bundles. Opticin binds heparan and chondroitin sulfates, suggesting that it may play a role in vitreoretinal adhesion. Similar to its role in articular cartilage, opticin may also stabilize vitreous gel structure by binding chondroitin sulfate proteoglycans.
Anatomy and Histology
Vitreous Body
Vitreous is a clear gel-like structure with a volume of about 4.0 mL. During invagination of the optic vesicle, the “primary” vitreous forms between the lens and the inner limiting membrane (ILM) of the retina. It is noteworthy that the ILM is continuous with Bruch’s membrane ( Fig. 23.3A ), demonstrating a common embryologic origin with analogous molecular composition and structure, suggesting important similarities later in life.

The “secondary” vitreous begins to develop at the 13-mm stage of embryogenesis and is derived from the retina and mesoderm of the hyaloid vascular system ( Fig. 23.3B ). Recent proteomic studies of human embryonic vitreous have identified changes in protein expression during the second trimester that may play a role in regression of the hyaloid vasculature. Confirmatory studies may thus open new avenues of drug development to prevent or treat pathologic neovascularization in the eye and elsewhere.
Classic depictions of human vitreous structure are shown in Fig. 23.4 . Modern concepts proposed membranous ( Fig. 23.5A ) and cisternal ( Fig. 23.5B ) systems. Sebag and Balazs performed dark-field slit microscopy to define the posterior vitreous cortex as a thin, membranous structure continuous from the ora serrata to the posterior pole. Two round holes are present in the prepapillary and premacular areas ( Fig. 23.6 ). Anteroposterior fibers ( Fig. 23.7 ) comprised of parallel collagen fibrils ( Fig. 23.8 ) arise from the vitreous base ( Fig. 23.9A ), where Gartner found “lateral aggregation” in older individuals. Vitreous base collagen fibers insert anterior to the ora serrata forming the anterior loop ( Fig. 23.9B ), important in anterior proliferative vitreoretinopathy (PVR). In the posterior pole, fibers extend through the premacular hole ( Figs. 23.6 and 23.7A ), but a few attach to the rim of the hole. Condensed bundles of fibers insert into the vitreous cortex in the midperiphery and equator ( Fig. 23.10 ).







Vitreoretinal Interface
The equatorial and posterior vitreoretinal interfaces consist of the posterior vitreous cortex, the ILM of the retina, and an intervening extracellular matrix.
Posterior Vitreous Cortex
The posterior vitreous cortex is 100–110 µm thick and consists of densely packed collagen fibrils ( Fig. 23.11 ). There is no vitreous cortex over the optic disc ( Figs. 23.6 and 23.7A ), and the cortex is thin over the macula. The prepapillary hole can sometimes be visualized clinically following posterior vitreous detachment (PVD). If peripapillary tissue is torn away during PVD and remains attached around the prepapillary hole, it is called a Vogt or Weiss ring. Hageman demonstrated a lamellar organization of the posterior vitreous cortex ( Fig. 23.12 ), confirmed in humans by three-dimensional optical coherence tomography (OCT) ( Fig. 23.13 ). During anomalous PVD (APVD), this lamellar structure predisposes to splitting along the potential cleavage planes between the lamellae, resulting in vitreoschisis.



Hyalocytes.
Hyalocytes are mononuclear cells embedded in the posterior vitreous cortex 20–50 µm from the ILM posteriorly ( Figs. 23.6 and 23.14 ). The highest density of hyalocytes is in the vitreous base followed by the posterior pole, with the lowest density at the equator. Balazs suggested that these cells synthesize vitreous HA, but Swann disagreed. Evidence suggests that hyalocytes maintain ongoing synthesis and metabolism of glycoproteins and may also synthesize collagen and enzymes.

The phagocytic capacity of hyalocytes has been described in vivo and demonstrated in vitro. Hyalocytes become phagocytic in response to inducting stimuli and are important in antigen processing and as initiators of the immune response, making it possible for intravitreal inoculation of antigens to promote systemic immunity. HA may have a regulatory effect on hyalocyte phagocytic activity. Various constituents of vitreous may be immunogenic and play a role in ocular inflammatory diseases. Sakamoto and Ishibashi have recently published excellent reviews of hyalocytes.
Hyalocytes are important in macular pucker when APVD and vitreoschisis leave these cells on the macula ( Fig. 23.15 ). Under the influence of cytokines, hyalocytes proliferate on the surface of the retina, resulting in hypercellular membranes. Hyalocytes also recruit cells from the circulation and the retina (glial cells) via the release of connective tissue growth factor and induce collagen gel contraction in response to platelet-derived growth factor and other cytokines, causing tangential vitreoretinal contraction.

Inner Limiting Membrane of the Retina
The ILM is a multilaminar structure of variable thickness topographically. Adjacent to Müller cell footplates is the lamina rara externa (0.03–0.06 µm) with no species variations or changes with topography or age. The lamina densa is thinnest at the fovea (0.01–0.02 µm) and thicker in the posterior pole (0.5–3.2 µm) than at the equator or vitreous base, where Foos found the ILM to be uniformly thin (51 nm) and the lamina rara to be 40 nm thick with traversing fibrils that were denser at sites corresponding to attachment plaques in Müller cells. The ILM is very thin over major retinal vessels ( Fig. 23.16 ) where defects allow glial cells to extend on to the inner retina. Acquired ILM defects are in the foveola, retinal pits, retinal tufts, and retinal lattice. The ILM progressively thickens posteriorly to about 306 nm at the equator and about 1887 nm posteriorly. Müller footplates are less numerous at the equator than at the vitreous base. Posteriorly, no Müller footplates were observed and the inner aspect of the ILM remains smooth, while the outer aspect is irregular. Peripherally, both inner and outer surfaces are smooth.

The ILM consists of type IV collagen, more abundant on the vitreous side of the ILM, and laminin, which is more abundant on the retinal side of the ILM ( Fig. 23.17 ). These are associated with glycoproteins, type VI collagen, which may contribute to vitreoretinal adhesion, and type XVIII, which binds opticin. Opticin binds to heparan sulfate, contributing to vitreoretinal adhesion. Type XVIII collagen also prevents cell migration from the retina into vitreous. Recent studies have also identified agrin, a basement membrane-associated heparan sulfate proteoglycan similar to collagen XVIII that provides proteolytically cleaved bioactive fragments for modulating cellular behavior at the vitreoretinal interface.

Intervening Extracellular Matrix
The interface between vitreous and adjacent structures consists of a complex formed by the vitreous cortex and basal laminae, which are firmly attached to their cells. The only part of vitreous not adjacent to a basal lamina is the annulus of the anterior vitreous cortex, which is directly exposed to the zonules and the aqueous humor of the posterior chamber, similar to the surface of articular cartilage, which is exposed to synovial fluid. Zimmerman and Straatsma claimed that there are fibrillar attachments between the posterior vitreous cortex and the ILM. The composition of these fibrillar structures is not known, and their presence has never been confirmed.
It is currently believed that an extracellular matrix “glue” of fibronectin, laminin, and other extracellular matrix components exists between vitreous and retina, causing adhesion to be fascial, as opposed to focal. Chondroitin sulfate is present at the sites of strong vitreoretinal adhesion such as the vitreous base and optic disc, forming the rationale for pharmacologic vitreolysis using avidin–biotin complex chondroitinase.
Topographic Variations
Strength of Vitreoretinal Adhesion.
Vitreous is attached to all contiguous structures, but is most firmly adherent at the vitreous base, which includes the posterior 2 mm of the pars plana and extends 1–4 mm posterior to the ora serrata, varying with age and topography (more posterior temporally). There are also topographic differences posteriorly, with greater adhesion at the posterior pole than the equator ( Fig. 23.18 ).

Peripheral Fundus and Vitreous Base.
The vitreous base is a three-dimensional structure that straddles the ora serrata. There is a high density of collagen fibrils oriented at right-angles to the inner surface of the ciliary epithelium and peripheral retina. The fibrils attach to the basement membrane of the nonpigmented epithelium of the pars plana and the ILM of the peripheral retina, intimately interwoven with the intervening extracellular matrix. Within the vitreous base, there are several anatomic variations where vitreoretinal adhesion is firm, adhesions that predispose to retinal breaks [see below]
Interface Along Major Retinal Blood Vessels.
The ILM thins and is sometimes absent over major retinal vessels ( Fig. 23.19 ). At such points, vitreous may be incarcerated into retina, directly continuous with perivascular tissue, attachments called “vitreoretino-vascular bands,” or “spider-like bodies,” purported to be vitreous fibrils that traverse the ILM and coil about retinal blood vessels. It is common for retinal vessels to be associated with paravascular rarefaction (cystic degeneration), retinal pits and tears, and avulsion of retinal vessels.

Retinal Sheen Dystrophy.
This ILM dystrophy has cystic spaces under the ILM and in the inner nuclear layer, and numerous areas of separation of the ILM from the retina with filamentous material ( Fig. 23.20 ). Endothelial cell swelling and degeneration, pericyte degeneration, and basement membrane thickening of retinal capillaries suggest that this condition is primarily a retinal vasculopathy with edema, swelling, and degeneration of Müller cells. Alternatively, the primary defect could be in Müller cells.

Vitreomacular Interface.
Attachment of vitreous to the macula occurs in an irregular, annular zone of 3–4 mm diameter, generally not visible by clinical examination in normal adults but possibly evident in fetal and young adult eyes and in pathologic conditions. The posterior vitreous cortex is thinner over the macula in a disc-shaped area about 4–5 mm in diameter. Discontinuity of the ILM in the fovea may be a site where glial cells extend onto the inner surface of the retina ( Fig. 23.21 ).

Vitreopapillary Interface.
At the rim of the optic disc the ILM ceases, although the basement membrane continues as the inner limiting membrane of Elschnig. This membrane is 50 nm thick and is believed to be the basal lamina of the astroglia in the optic nerve head. At the centralmost portion of the optic disc the membrane thins to 20 nm, follows the irregularities of the underlying cells of the optic disc, and is composed only of glycosaminoglycans and no collagen (central meniscus of Kuhnt). Given that the ILM prevents the passage of cells, the thinness and chemical composition of the central meniscus of Kuhnt and the membrane of Elschnig may account for frequent cell proliferation from or near the optic disc.
Vitreous attachment to the optic disc may persist even though the vitreous is detached elsewhere ( Fig. 23.22 ). This adhesion may be fortified by epipapillary membranes. The entire complex may subsequently detach, resulting in a ring (Weiss) of tissue composed of fibrous astrocytes and collagen ( Fig. 23.23 ) that flutters in and out of the visual axis, causing “floaters.” Vitreopapillary adhesion contributes to macular holes and any vitreomaculopathy that features intraretinal cystoid spaces, presumably due to the influence of vitreopapillary adhesion upon the vectors of tangential traction at the macula.


Physiology
Biochemical
Vitreous is important in maintaining transparency for maximal photon transmission to the retina. Vitreous may also maintain lens transparency by mitigating the effects of reactive oxygen species on lens proteins and thus preventing cataracts. This antioxidant effect is primarily the result of high concentrations of ascorbate in vitreous, an observation originally made in 1944 by Friedenwald and colleagues.
Gisladottir et al. recently emphasized the influence of vitreous on various physiologic processes and showed that vitrectomy can have considerable effects, both beneficial and harmful. Vitrectomy reduces the risk of retinal neovascularization, but increases the risk of iris neovascularization, reduces macular edema, but stimulates cataract formation. In fact, one approach to mitigating cataract formation following vitrectomy is to leave 3–4 mm of retrolental vitreous intact.
Biophysical
During ocular saccades, the rotational force of the eye wall is transmitted to the vitreous body via attachments to adjacent structures. During both acceleration and deceleration phases of saccades, vitreous movement lags behind the eye wall, resulting in markedly reduced acceleration. This “slack and lag” results from vitreous viscoelasticity, dampening the force at any given internal vitreous attachment. The inferonasal displacement of the optic disc and the shorter distance between the disc and ora inferiorly and nasally make the relief of torsional strain on equatorial and anterior vitreoretinal attachments greater nasally and inferiorly. Accordingly, the greatest torsional strain on anterior and equatorial vitreous attachments should occur during lateral saccades, with the point of maximum strain located somewhere in the superotemporal quadrant, the site of most frequent retinal tears.
Pathology
Age-Related Vitreous Degeneration
Liquefaction
After age 40 years there is a significant decrease in the gel volume and a concurrent increase in the liquid volume of vitreous, primarily centrally. In the posterior vitreous such changes form pockets of liquid vitreous, called “lacunae” ( Fig. 23.24 ). When a single, large pocket forms, the terms “bursa” or “precortical pocket” have been employed. The large posterior lacuna that is present in young individuals is the bursa premacularis, as originally described by Jan Worst and now visualized on Swept Source OCT imaging. Indeed, Balazs and Denlinger found evidence of liquid vitreous after the age of 4 years and observed that, by the time the human eye reaches adult size (ages 14–18 years), 20% of the total volume is liquid vitreous. By the age of 80–90 years more than half the vitreous body is liquid, which can collect in lacunae throughout or a ‘pocket’ posteriorly, a pocket, sometimes referred to as the posterior precortical vitreous pocket ( Fig. 23.24 ).

Pathogenesis of Vitreous Liquefaction.
Changes in collagen or the conformation of HA with subsequent crosslinking and aggregation of fibrils into bundles may result in vitreous liquefaction. Free radicals generated by metabolism and/or photons alter vitreous macromolecules and trigger dissociation of collagen from HA, leading to liquefaction. Vitreous liquefaction may also result from changes in the minor glycosaminoglycans and chondroitin sulfate profile of vitreous. Such mechanisms may be at play in myopic vitreopathy where liquefaction occurs unrelated to aging.
Biochemical studies support the age-related rheologic observations described above. Total vitreous collagen content does not change after age 20–30 years. However, collagen concentration in gel vitreous at the ages of 70–90 years (0.1 mg/mL) was significantly greater than at the ages of 15–20 years (0.05 mg/mL; p <.05). Since the total collagen content does not change, this is likely due to the decreased volume of gel vitreous that occurs with aging and a consequent increase in the concentration of the collagen in the remaining gel. This concept is supported by the finding that vitreous HA concentration increases until about the age of 20, when adult levels are attained. Thereafter, until 70 years, there are no changes in the HA concentrations of either the liquid or gel compartments. This necessarily means that there is an increase in the HA content of liquid vitreous and a concomitant decrease in the HA content of gel vitreous, since the amount of liquid vitreous increases and the amount of gel vitreous decreases with age.
Structural Changes With Aging
Aging of the Vitreous Body.
The aforementioned rheologic and biochemical alterations induce significant structural changes during aging, consisting of a transition from a clear gel in youth (see Fig. 23.2 ) to a fibrous structure in adults (see Fig. 23.7A ). In old age there is advanced liquefaction with thickening and tortuosity of vitreous fibers, and collapse of the vitreous body ( Fig. 23.25 ). Postmortem studies found such changes in 70% of subjects in the eighth decade. Gel liquefaction occurs earlier and is more extensive in myopic eyes, and is accelerated with inflammation, trauma, and arthro-ophthalmopathies.

Aging of the Vitreoretinal Interface.
With aging there is significant increase in ILM thickness ( Fig. 23.26 ). This may play a role in weakening of vitreoretinal adhesion with age, although the mechanism of such a phenomenon is presently unclear.

Peripheral Fundus.
Teng and Chi found that the width (in the radial dimension) of the vitreous base posterior to the ora serrata increases with age to over 3.0 mm. There is also posterior migration of the posterior border of the vitreous base with age, mostly temporally. In addition, Gartner found “lateral aggregation” of the collagen fibrils in the vitreous base of older individuals. These and the changes listed below play important roles in the pathogenesis of peripheral retinal breaks and rhegmatogenous retinal detachmnent:
Ora bays ( Fig. 23.27 )
Fig. 23.27
A meridional complex ( arrow ) and dentate process ( arrowhead ) are located at the margin of a non-enclosed ora bay.
Meridional folds ( Fig. 23.28 )
Fig. 23.28
Meridional folds, one in line with a meridional complex ( arrowhead ) and the other ( arrow ) in line with a meridional complex and enclosed ora bay ( asterisk ).
(Reproduced with permission from Green WR. Pathology of the retina. In: Frayer WC, editor. Lancaster course in ophthalmic histopathology, unit 9. Philadelphia: FA Davis; 1981.)
Meridional complexes ( Figs. 23.27 and 23.29 )
Fig. 23.29
Peripheral retinal excavation ( arrowhead ) in line with a meridional complex and an enclosed ora bay.
(Reproduced with permission from Green WR. Pathology of the retina. In: Frayer WC, editor. Lancaster course in ophthalmic histopathology, unit 9. Philadelphia: FA Davis; 1981.)
Peripheral retinal excavations ( Fig. 23.29 )
Retinal tufts
Noncystic retinal tufts ( Fig. 23.30 )
Fig. 23.30
Noncystic peripheral retinal tuft. Most tufts consist of glial cells, and some have strands of vitreous attached ( arrow ) (hematoxylin and eosin, ×215).
(Reproduced with permission from Green WR. Retina. In: Spencer WH, editor. Ophthalmic pathology: an atlas and textbook, vol. 2. Philadelphia: WB Saunders; 1985.)
Cystic retinal tufts ( Fig. 23.31 )
Fig. 23.31
Cystic retinal tuft. (A) The lesion consists of an area of retinal thickening caused by cystic changes ( asterisks ) and glial cell proliferation (hematoxylin and eosin, ×220). (B) Scanning electron microscopy (EM) demonstrates that the tuft is a cystoid formation of fibers, similar to those of the nerve fiber layer, and cells, similar to those found in the inner plexiform layer of the retina, that are connected to the inner limiting membrane of the retina. This scanning EM shows the insertion of the vitreous collagen fibers on the tuft’s apical surface.
(Panel B reproduced with permission from Dunker S, Glinz J, Faulborn J. Morphologic studies of the peripheral vitreoretinal interface in humans reveal structures implicated in the pathogenesis of retinal tears. Retina 1997;17:124–30.)
Zonular traction tufts ( Fig. 23.32 )
Fig. 23.32
Zonular traction tuft. Gross (A) and microscopic (B) appearances of zonular tractional tuft ( arrow in panel A) composed of a strand of glial cells whose anterior part consists of two layers of embryonal-like epithelium ( arrows in panels B and C; hematoxylin and eosin, ×65). (C) Higher magnification (hematoxylin and eosin, ×290).
(Panels A and B reproduced with permission from Green WR. Pathology of the retina. In: Frayer WC, editor. Lancaster course in ophthalmic histopathology, unit 9. Philadelphia: FA Davis; 1981. Panel C reproduced with permission from Green WR. Retina. In: Spencer WH, editor. Ophthalmic pathology: an atlas and textbook, vol. 2. Philadelphia: WB Saunders; 1985.)
Spiculate and nodular pigment epithelial hyperplasia ( Fig. 23.33 )
Fig. 23.33
Hyperplasia of pigment epithelium at ora serrata. Hyperplastic pigment epithelium ( arrow ) extends into the vitreous cavity at the ora serrata (periodic acid–Schiff; ×220).
(Reproduced with permission from Green WR. Pathology of the retina. In: Frayer WC, editor. Lancaster course in ophthalmic histopathology, unit 9. Philadelphia: FA Davis; 1981.)
Retinal lattice ( Figs. 23.34–23.38 )
Fig. 23.34
Paravascular retinal lattice. Radial perivascular lattice with sclerotic vessel and hyperplastic retinal pigment epithelium, with migration into the retina in a paravascular location.
Fig. 23.35
Lattice wicker. Gross appearance of retinal lattice, with sclerotic vessels that have a wicker appearance.
Fig. 23.36
Histopathology of retinal lattice. Lattice lesion with retinal thinning, discontinuity of inner limiting membrane ( arrows ), pocket of fluid vitreous ( asterisk ), and glial cell proliferation in vitreous at margin ( arrowheads ) (Alcian blue stain; ×145).
Fig. 23.37
Trypsin digestion preparation of retina discloses relatively acellular capillaries in area of lattice degeneration (right) (hematoxylin and eosin, and periodic acid–Schiff stains; ×185).
Fig. 23.38
Retinal tear at posterior margin of area of retinal lattice.
White with pressure, white without pressure
Verruca ( Fig. 23.39 ).
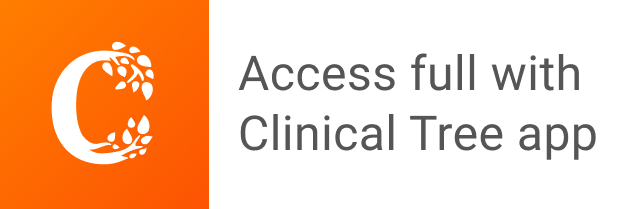