Introduction
The vitreous body is the largest single structure of the human eye, comprising approximately 80% of the eye’s volume ( Fig. 6.1 ). Anteriorly, it is delineated by and adjoins the ciliary body, the zonules, and the lens. Posteriorly, the vitreous body is adjacent to the retina and optic disc. Vitreous has important physiologic functions that including providing structural support, serving as a diffusion barrier between the anterior and the posterior segments of the eye, providing metabolic buffering against oxidative stress, and maintaining optical transparency. This chapter focuses on those aspects of vitreous physiology and pathophysiology that have clinical importance, by reviewing relevant biochemistry, anatomy, and biophysics.

Biochemistry
Vitreous is an extracellular matrix in the form of a clear viscous gel that is composed of 98% water; 15% to 20% of the water is bound to glycosaminoglycans, primarily hyaluronan (HA). The gel structure of vitreous results from the arrangement of long, nonbranching collagen fibrils integrated in a network of HA, which stabilizes the gel structure and the conformation of the collagen fibrils ( Fig. 6.2 ). Changes in vitreous HA and collagen alter the viscosity of the gel and destabilize the vitreous body. In myopia and aging, this contributes to posterior vitreous detachment which can result in retinal detachment, when anomalous. Persistent attachment to the retina can exacerbate diabetic retinopathy and age-related macular degeneration (see further in chapter).

Glycosaminoglycans
Glycosaminoglycans are polysaccharides with repeating disaccharide units that are characteristic for each glycosaminoglycan. The relative amounts and molecular sizes of various glycosaminoglycans are tissue specific. Three major glycosaminoglycans of the vitreous body are hyaluronan, chondroitin sulfate, and heparan sulfate.
Hyaluronan
HA was initially termed hyaluronic acid due to its colorless nature (“hyalos” meaning glass in Greek) and its composition that includes uronic acid. In 1986 this term was replaced with “hyaluronan” to be more consistent with nomenclature conventions for polysaccharides and to reflect that HA can be found in either its protonated acid form (hyaluronic acid) or base salt form (sodium hyaluronate) based on the surrounding pH. HA plays an essential role in many physiologic processes. Importantly for vision, HA promotes transparency via its anti-inflammatory and anticicatricial (scarring) properties.
HA is a long, unbranched polymer of repeating D-glucuronic acid and N-acetyl-D-glucosamine that forms a linear threefold helix. , It is unique as a glycosaminoglycan in that it is nonsulfated. It can assume vastly different configurations and volumes depending on its environment, particularly if it is surrounded by water or ionic charges. The volume of nonhydrated HA is 0.66 cm 3 /g, in contrast with the volume of its hydrated form of 2000 to 3000 cm 3 /g. When surrounded by high ionic charges, the charged HA molecule can contract, thus resulting in contraction of the vitreous body and tractional forces on adjacent ocular structures, such as the retina. The HA molecule forms large, open coils with its anionic sites spread apart. This arrangement of small-diameter fibers separated by highly hydrated glycosaminoglycan chains permits the transmission of light to the retina with minimal scattering. Furthermore, these open coils can become highly entangled in the vitreous body with many electrostatic interactions. These interactions can influence osmotic pressure, ion transport and distribution, electric potentials within the vitreous, and vitreous collagen fibril assembly. ,
Hyaluronan-collagen binding
Type II collagen binds to HA with a binding affinity of 755 nM. It has been proposed that HA stabilizes vitreous gel via long-range spacing, but studies using HA lyase found that the gel structure was not destroyed. Vitreous collagen fibrils are believed to form a crosslinked polymer system with HA, with collagen providing structural support, and osmotically active HA adding bulk to the gel.
Hyaluronan lipid binding
In addition to collagen, HA might bind to vitreous phospholipids, thereby disrupting their physiologic interaction with collagen and promoting undesirable vitreous liquefaction. Based on the binding affinity of HA and phospholipids, however, only about 4% of the hydrophobic hydrogens of the HA molecules in human vitreous would be expected to bind to phospholipids; thus, it is unclear whether this amount of binding has significant effects on vitreous liquefaction ( Fig. 6.3 ). Interestingly, diabetic patients who have earlier than expected vitreous liquefaction were found to have higher vitreous levels of lipids. , This could contribute to exacerbation of diabetic retinopathy (see further in chapter). As a corollary, it might be interesting to determine phospholipid levels in myopic eyes that have precocious vitreous liquefaction to determine if HA binding to phospholipids might play a role in disrupting normal vitreous physiology.

Chondroitin sulfate
Vitreous contains two chondroitin sulfate proteoglycans. The minor type is type IX collagen. The major type is versican, which forms complexes with HA and microfibrillar proteins such as fibulin-1 and fibulin-2. Chondroitin sulfate protects against vitreous liquefaction by preventing collagen degradation, thus preserving gel vitreous structure.
Heparan sulfate
Heparan sulfate is a sulfated galactosamine-containing glycosaminoglycan present in vitreous in small amounts. It is thought to be important in maintaining transparency by ensuring an adequate distance between vitreous collagen fibrils.
Vitreous collagens
Vitreous collagen fibrils are thin, with diameters of approximately 10 to 20 nm. They are composed primarily of type II collagen (75%) surrounding a central core of a hybrid of types V/XI (10%), with chondroitin sulfate side-chains of type IX collagen (15%) located on the surface of the fibril ( Fig. 6.4 ). At physiologic pH, vitreous collagen undergoes fibrillization with crosslinkages between lysyl and hydroxylysyl residues, catalyzed by copper-dependent lysyl oxidase. This fibrillization and crosslinkage adds tensile strength and mechanical stability. At higher ionic strengths, collagen fibrils increase in size and decrease in concentration, which may be relevant to the pathophysiology of vitreous collagen aggregation during age-related fibrous liquefaction.

Type II collagen
Type II collagen is the most prevalent type of collagen found in human vitreous. It is composed of three identical alpha chains that form a triple helix. This helix is stabilized by hydrogen bonds between opposing residues in different chains. , Although the cell type responsible for vitreous collagen synthesis is not known (retinal Müller cells, hyalocytes, and ciliary body epithelial cells are candidates), type II collagen is first synthesized as a soluble procollagen and excreted into the extracellular space, where it is then cleaved by N- proteinase and C- proteinase enzymes. The resulting collagen molecule is reduced in solubility and able to form fibrils through covalent crosslinkages. On the surface of the fibril are N -propeptides, which bind growth factors such as transforming growth factor (TGF)-β 1 and bone morphogenic protein-2. This affinity for growth factors may contribute to proliferative vitreo-retinal disorders. Additionally, alternative splicing of type II collagen propeptides is important in the pathogenesis of hereditary vitreo-retinopathies, most commonly Stickler syndrome, which is the most frequent inherited cause of retinal detachment.
Type IX collagen
Type IX collagen is a disulfide bonded heterotrimer that is secreted as a mature collagen and does not undergo processing prior to incorporation onto the surfaces of the major collagen fibrils. It contains both collagenous and noncollagenous regions, as well as a covalently linked chondroitin sulfate glycosaminoglycan chain, allowing the molecule to assume a proteoglycan form. Type IX collagen modulates the spatial arrangement of collagen fibrils by both bridging together and spacing apart individual fibers. Age-related changes in type IX collagen may play an important role in the age-related aggregation of vitreous collagen fibrils. There are also reports of mutations in type IX collagen causing ocular manifestations in some forms of Stickler syndrome. ,
Type V/XI collagen
Collagen types V and XI are found complexed into a “hybrid” configuration located in the core of the major vitreous collagen fibrils ( Fig. 6.4 ). These collagens are secreted as procollagens with subsequent processing removing the C-propeptide and partially removing the N-propeptide. The hybrid type V/XI collagen forms heterotypic fibrils, with type V collagen playing an essential role in the initiation of collagen fibril formation. This suggests that hybrid V/XI collagen could also play an important role in vitreous fibril assembly.
Type VI collagen
Type VI collagen is found in small amounts within the vitreous body. It can bind both type II collagen and HA, suggesting a potential role for organizing the supramolecular structure of vitreous. It may also play a role in promoting vitreo-retinal adhesion because type VI collagen has been identified at the vitreo-retinal interface and is known to bind vitreous type II collagen, as well as type IV collagen found in the inner limiting membrane of the retina.
Type VII collagen
Type VII collagen has been found at the vitreo-retinal interface, suggesting a role in mediating vitreo-retinal adhesion.
Non-collagenous structural proteins
Fibrillins
Fibrillin-1 and fibrillin-2 are found in vitreous, but it is unknown whether these microfibrils contribute significantly to vitreous structure. Mutations in fibrillin-1 cause Marfan syndrome, which features ocular manifestations of lens dislocation, myopia, and rhegmatogenous retinal detachment.
Fibulins
Fibulins are a family of extracellular matrix glycoproteins that are thought to have bridging roles in extracellular matrix assembly, including interactions with versican and fibronectin. However, it is not known whether fibulins contribute significantly to the structural stability of vitreous.
Versican
Versican is a large chondroitin sulfate proteoglycan with an N-terminal domain that binds to HA. Mutations in the gene encoding versican can cause “ versican vitreo-retinopathy ” with retinal detachment and dystrophy. Wagner syndrome of erosive vitreo-retinopathy is a hereditary condition with vitreous abnormalities caused by splice site mutations in the versican gene. ,
Opticin
Opticin is a small leucine-rich repeat proteoglycan that is bound to the surface of heterotypic collagen fibrils and is expressed by nonpigmented ciliary epithelium. Opticin has antiangiogenic properties by coating collagen fibrils and weakening integrin-mediated endothelial cell adhesion to collagen, thereby inhibiting integrin-mediated signaling required for angiogenesis. Opticin also plays a role in preventing the aggregation of collagen fibrils in vitreous, thus promoting transparency.
Supramolecular organization
Vitreous collagen fibrils are widely spread apart with significant amounts of proteoglycans between fibrils. The average mesh size through which endogenous or exogenous materials can diffuse through the vitreous body is 550 ± 50 nm. The surface of the fibril has N-propeptide extensions of the hybrid V/XI collagen and type IX collagen with extensions of its chondroitin sulfate chains, which mediate interactions with other extracellular matrix molecules. Throughout the vitreous body, collagen fibrils interpenetrate complexes of HA molecules, forming a crosslinked polymer system. Studies have shown that if collagen is removed, the remaining HA forms a viscous solution, whereas if HA is removed, the gel shrinks. This suggests that collagen fibrils provide structure to the vitreous, which is then “inflated” by the osmotic contribution of hydrophilic HA. Both collagen and HA contribute to vitreous viscoelasticity. Collagen, HA, and other proteoglycans all work synergistically to maintain vitreous stiffness, with HA as the key mediator of vitreous adhesivity.
Dissolved substances
Dissolved substances in the vitreous body are inorganic and organic compounds as shown in Table 6.1 , where serum values are given for comparison.
Vitreous | Serum | |
---|---|---|
Sodium (mmol/L) | 146.7 | 139.7 |
Potassium (mmol/L) | 5.73 | 4.25 |
Chloride (mmol/L) | 121.6 | 104.4 |
Calcium (mmol/L) | 1.128 | 2.368 |
Magnesium (mmol/L) | 0.900 | 0.871 |
Glucose (mmol/L) | 2.97 | 6.16 |
Lactate (mmol/L) | 3.97 | 0.84 |
Copper (μmol/L) | 0.519 | 16.35 a |
Zinc (μmol/L) | 1.95 | 9.73 a |
Selenium (μmol/L) | 0.1035 | 1.00 a |
Iron (μmol/L) | 3.11 | 19.95 a |
Ferritin (μg/L) | 19.5 | 101 b |
Transferrin (g/L) | 0.0878 | 0.2394 b |
a Data from Baudry J, Kopp JF, Boeing H, Kipp AP, Schwerdtle T, Schulze MB. Changes of trace element status during aging: Results of the EPIC-Potsdam cohort study. Eur J Nutr . 2020;59(7):3045–3058.
b Data from National Report on Biochemical Indicators of Diet and Nutrition in the U.S. Population 1999–2002 . Department of Health and Human Services, Centers for Disease Control and Prevention; 2008:1–155. Accessed January 5, 2022. https://www.cdc.gov/nutritionreport/99-02/pdf/nutrition_report.pdf .
Vitreous plasma gradients
Gradients of salts and organic substances exist in both directions between vitreous and plasma ( Table 6.1 ). These gradients result from several mechanisms: blood-aqueous and blood-retinal barriers, metabolism in the retina and ciliary body, and diffusion through the vitreous body, especially for small molecules.
Regional differences
Oxygen tension is lower in the center of the vitreous body, due to oxygen flux from the retina toward vitreous corresponding to arterioles; the flux goes in the opposite direction corresponding to venules. , Glucose concentration is highest in the center, followed by the posterior vitreous body, with the lowest concentrations anteriorly. Lactate is found at highest concentrations in the posterior vitreous, with decreasing concentrations more anteriorly.
Anatomy
The vitreous body
The mature vitreous body is a transparent gel ( Fig. 6.1 , right) occupying the center of the eye. It is almost spherical, except for the anterior part, which is concave, corresponding to the presence of the crystalline lens. The mean vitreous chamber volume is 4.65 ± 0.43 mm 3 for women and 4.97 ± 0.47 mm 3 for men, with a significant positive correlation with axial length, and a negative correlation between age and vitreous (gel) volume. Histologic studies of vitreous structure introduce artifacts owing to specimen dehydration; thus, dark-field slit microscopy has been instrumental in the study of vitreous anatomy ( Fig. 6.5 ).

Anterior and posterior vitreous cortex
The outermost part of the vitreous, called the cortex (not “ hyaloid ,” which is a term that should only be used to refer to the embryonic artery), is divided into an anterior cortex (“hyaloid face”) and a posterior cortex, the latter being approximately 100-μm thick. The cortex consists of densely packed collagen fibrils. Vitreous base collagen fibers insert anterior to the ora serrata (linear junction between the retina and the pars plana ciliaris) forming the anterior loop. In youth, the peripheral and posterior vitreous cortex can be seen as a thin, membranous structure that is continuous from the ora serrata to the posterior pole with greater light scattering than in the central vitreous ( Fig. 6.6 ; Fig. 6.7 , top panel). By middle age, there are visible fibers that arise from the vitreous base and course continuously to the posterior pole of the fundus ( Fig. 6.7 , middle panel). In addition to vitreous fibrils, there are larger fibers oriented perpendicular to the retina that insert into the extracellular matrix between the retina and vitreous body. By old age, these fibers become aggregated and tortuous, associated with liquid vitreous ( Fig. 6.7 , bottom panel).


Hyalocytes
Hyalocytes are mononuclear cells embedded in the posterior vitreous cortex ( Fig. 6.8A ) anterior to the inner limiting membrane (ILM) of the retina ( Fig. 6.8C ). Although there are similarities with microglia due to a common embryologic origin, microglia are cells of the retina, while hyalocytes are cells of the vitreous body. , Sized about 10 to 15 μm in diameter, hyalocytes are oval-, spindle-, or star-shaped, depending upon their state of activity, (i.e., dormant, activated, phagocytosing, migrating, etc). , The highest density of hyalocytes is near the vitreous base, followed by the posterior pole, with the lowest density at the equator of the ocular fundus. , Utilizing staining and confocal imaging modalities, hyalocytes have historically been studied ex vivo ( Fig. 6.8A,B ). But recent technologic advances have enabled hyalocyte imaging in vivo via B-scan optical coherence tomography (OCT) ( Fig. 6.8C ) and adaptive optics scanning light ophthalmoscopy (AO-SLO) in the coronal plane ( en face ) ( Fig. 6.8D , top). Such studies in humans revealed hyalocytes in the temporal retina and near the optic disc with regular spatial separation in healthy subjects, which contrasted with nonuniform distribution and altered morphology in patients with retinopathies. , Coronal plane imaging in vivo has also led to the observation that these cells migrate over time ( Fig. 6.8D , bottom).

Physiologic functions of hyalocytes include phagocytosis and antigen processing, as well as recruitment of circulating monocytes in response to antigens. , Transcriptional analysis has shown strong gene expression for processes related to antigen processing, presentation, and immune modulation, including the SPP1 , FTL , CD74 , and HLA-DRA genes. Compared with other myeloid cell lines, the main differences in hyalocytes were the significantly increased expression of genes related to immune privilege of the eye, including POMC , CD46 , and CD86 . Other potential hyalocyte physiologic functions relate to metabolism, including ongoing synthesis and metabolism of glycoproteins, as well as possible synthesis of collagen and enzymes. , Hyalocytes have been found to be located in the regions with the highest concentration of HA, suggesting they may be responsible for vitreous HA synthesis. Enzymes for HA synthesis have been found within hyalocytes, and hyalocytes have been shown to take up and internalize labeled intermediates for HA synthesis. , In turn, HA may have a regulatory effect on hyalocyte phagocytic activity.
Pathologically, hyalocytes can induce collagen gel contraction in response to platelet-derived growth factor and other cytokines, causing tangential vitreo-retinal traction. , Hyalocytes might also play a role in retinal neovascularization. Boneva et al. analyzed samples of retinal neovascularization from patients with proliferative diabetic retinopathy and found increased M2 macrophages within fibrovascular membranes, postulated to be derived from vitreous hyalocytes. This hypothesis was supported by the staining of cultivated vitreous hyalocytes, which revealed a strong expression of α-smooth muscle actin (α-SMA). As α-SMA is a marker for myofibroblasts, these results suggest that hyalocytes can differentiate into myofibroblasts and might therefore comprise part of the fibrovascular membrane in neovascularization.
Vitreous base
The vitreous base is a three-dimensional zone, best conceived as a “doughnut” or “bagel” straddling the ora serrata. It extends from approximately 2-mm anterior to the ora serrata to about 3-mm posterior to the ora serrata, and it is several millimeters thick. Collagen fibrils are densely packed in this region, inserting anterior and posterior to the ora serrata ( Fig. 6.9 , left). Fibers can be seen within the vitreous base that course over the ora serrata, forming the “anterior loop” ( Fig. 6.9 . right), an important structure in anterior proliferative vitreo-retinopathy.

The vitreo-retinal interface
The vitreo-retinal interface is defined as the fascial (sheet-like) apposition of the outer surface of the posterior vitreous cortex and the inner surface of the ILM of the retina ( Fig. 6.10 ).

Inner limiting membrane of the retina
The ILM is 1- to 3-μm thick, consisting of type IV collagen (more abundant on the vitreous side) and laminin (more abundant on the retinal side) ( Fig 6.10B ). There are also glycoproteins, type VI collagen, which may contribute to vitreo-retinal adhesion, and type XVIII collagen, which binds opticin and prevents cell migration from the retina into the vitreous body. , The ILM is multilayered and can be considered the basal lamina of Müller cells, the foot processes of which insert into the interface ( Fig. 6.11 ). Adjacent to Müller cell footplates is the lamina rara externa that is 0.03- to 0.06-μm thick with no changes related to topography or age. The lamina densa (central lamella) is thinnest at the fovea (0.01–0.02 μm).

Posterior vitreous cortex/inner limiting membrane adhesion
The vitreous cortex is firmly attached to the ILM in the vitreous base region, around the optic disc (Weiss ring), along retinal blood vessels, and in the area surrounding the fovea to a diameter of 500 μm. , There is no vitreous cortex over the optic disc, and the cortex is thin over the macula. Vitreo-retinal adhesion is strong in young individuals, and dissection of the retina from vitreous often leaves inner retinal tissue adherent to the vitreous cortex. , , , ( Fig. 6.11 ) Under pathologic conditions, the strong adhesion between the posterior vitreous cortex and the retinal ILM plays an important role, as is discussed next.
Intervening extracellular matrix
The interface between vitreous and adjacent structures consists of a complex formed by the outer vitreous complexed with basal laminae, which are firmly attached to their cells. It is currently believed that an extracellular matrix “glue” of fibronectin, laminin, and other extracellular matrix components exists between vitreous and retina, causing adhesion to be fascial (sheet-like), as opposed to focal or linear. , , Chondroitin sulfate is present at the sites of strong vitreo-retinal adhesion such as the vitreous base and optic disc, forming the rationale for pharmacologic vitreolysis using avidin-biotin complex chondroitinase. ,
Topographic variations
Strength of vitreo-retinal adhesion
Vitreous is attached to all contiguous structures but is most firmly adherent at the vitreous base. There are also topographic differences posteriorly, with greater adhesion at the posterior pole than the equator.
Peripheral fundus and vitreous base
There is a high density of collagen fibrils oriented at right angles to the inner surface of the ciliary epithelium and peripheral retina. Vitreous fibrils attach to the basement membrane of the nonpigmented epithelium of the pars plana portion of the ciliary body and the ILM of the peripheral retina, interwoven with the intervening extracellular matrix. Within the vitreous base, there are several anatomic variations where vitreo-retinal adhesion is firm, predisposing to peripheral retinal breaks.
Interface along major retinal blood vessels
The ILM thins and is sometimes absent over major retinal vessels. , At such points, vitreous may be embedded within the retina, directly continuous with perivascular tissue. These form attachments called “vitreo-retinovascular bands” or “spider-like bodies,” that are vitreous fibrils traversing the ILM to coil about retinal vessels. ,
Vitreo-macular interface
Attachment of vitreous to the macula occurs in an irregular, annular zone of 3- to 4-mm diameter, generally not visible by clinical examination in normal adults but possibly evident in fetal and young adult eyes and in pathologic conditions. The posterior vitreous cortex is thinner over the macula in a disc-shaped area about 4 to 5 mm in diameter. Discontinuity of the ILM in the fovea may be a site where glial cells extend into the vitreo-retinal interface.
Vitreo-papillary interface
The ILM ceases at the rim of the optic disc, although the membrane continues as the inner limiting membrane of Elschnig. This 50-nm thick membrane is believed to be the basal lamina of the astroglia in the optic nerve head. At the central-most portion of the optic disc the membrane thins to 20 nm, follows the irregularities of the underlying cells of the optic disc, and is composed only of glycosaminoglycans and no collagen (central meniscus of Kuhnt). Given that the ILM prevents the passage of cells, the thinness and chemical composition of the central meniscus of Kuhnt and the membrane of Elschnig may account for frequent cell proliferation from or near the optic disc.
Vitreous attachment to the optic disc may persist even though vitreous is detached elsewhere. Vitreo-papillary adhesion may be fortified by epipapillary membranes. , The entire complex may subsequently detach, resulting in a Weiss ring which can cause floaters, and when extensive, results in vision degrading myodesopsia .
Vitreous biophysics
Transvitreous transport
As mentioned previously, the average mesh size through which endogenous or exogenous materials can diffuse is 550 ± 50 nm. Intravitreal drug injection is one of the most common ophthalmic procedures performed to introduce exogenous substances for medicinal purposes. Pharmacotherapy via intravitreal injection would theoretically be greatly improved if more were known about how substances traverse vitreous to access the fundus. Unfortunately, there is a paucity of such information. Furthermore, there is no consideration given to controlling the location of drug injection during clinical treatments. It stands to reason that drug distribution will be very different if introduced into an area of gel vitreous as opposed to a pocket of liquid vitreous, called a lacuna ( Fig. 6.12 ). Assuming a homogeneous structure in the vitreous body, (which is probably not true because common conditions like myopia, diabetes, and aging substantially alter vitreous structure), injected substances may move through the vitreous body by two different processes: diffusion or convective flow. Both diffusion and convective flow are inversely related to vitreous viscosity.

Diffusion
Transvitreous diffusion has been examined in humans using fluorescein as a tracer to measure the biophysical behavior of the gel during vitreous fluorophotometry ( Fig. 6.13 ).Although this testing modality generated considerable interest in the past, there have never been clinical applications that resulted in enhanced care. One possible reason is that the models do not take into consideration the profound heterogeneity in vitreous structure with age, myopic vitreopathy, and diabetic vitreopathy (see further in chapter), which are likely to impact the physiology of transvitreous diffusion and the pharmacokinetics and pharmacodynamics of drugs injected into the vitreous body. It is generally believed that diffusion is the primary mechanism of drug delivery during intravitreal pharmacotherapy. However, this is likely to be an oversimplification because the distribution of a particular molecule in the vitreous gel versus time occurs according to the diffusion properties of that molecule. The mesh size of bovine vitreous has been estimated to be about 500 nm, which is much larger than the biologics typically used in intravitreal injections (typically <10 nm). , Thus, vitreous is believed to allow relatively unrestricted diffusion for smaller particles. However, the diffusion of positively charged molecules is restricted in vitreous, due to the overall negative charge of the vitreous body. Liquefaction (myopia, diabetes, aging, or vitrectomy) causes the viscosity of vitreous to approach that of water promoting modest increase in particle diffusion. This is likely significant for larger molecules, although the effects of vitreous liquefaction may be less significant for smaller drug molecules given that the mesh size prior to liquefaction already does not restrict diffusion. The presence of ocular barriers to the systemic circulatory system is the probable explanation for why transient changes in systemic circulating drug levels are reflected slowly in the vitreous body.

The rate of change of the vitreous body concentration of various substances, most notably potassium, can be used in forensic medicine to rapidly and reliably assist with postmortem time of death determination. , Postmortem analyses can also reveal electrolyte abnormalities and hyperglycemia, which can help elucidate contributing causes of death. Zilg et al. found that samples from the left vs. right eye, and center vs. whole vitreous samples, all gave similar results. The time constants for many substances are of the same magnitude as those describing glucose transport between blood and brain; that is, half of the maximum is achieved in approximately 10 minutes.
Convective flow
In addition to diffusion, molecules can move through the vitreous via convective flow, also referred to as bulk flow. Convective flow occurs as aqueous permeates posteriorly through the vitreous body. Low-molecular-weight substances move faster, diffusing in all directions, and are virtually unaffected by convective flow. However, high-molecular-weight substances move through the vitreous body more via convective flow. , The velocity of posteriorly directed convective fluid flow in rabbit eyes has been estimated at 2 × 10 −5 cm/min.
Half-lives of intravitreal drugs range from less than 10 hours for small molecule drugs, to around 1 week for biologics. Drug elimination from the vitreous body can occur via anterior elimination through aqueous humor outflow and posterior elimination via the blood-ocular barriers, which consist of tight junctions with a pore size of about 2 nm. The permeability of the blood-ocular barriers appears to be the rate-limiting step for smaller molecules, with small lipophilic drugs exhibiting higher rates of clearance. , There are conflicting reports in the literature about whether biologics are primarily eliminated via the anterior or posterior pathway, but more recent modeling studies and findings of rabbit and human studies of bevacizumab concentrations in aqueous humor indicate that proteins such as anti-vascular endothelial growth factor (anti-VEGF) molecules are likely mainly eliminated via the anterior pathway. , Strategies to reverse this might increase drug levels at the macula and possibly improve efficacy, as well as decrease the need for frequent reinjection. Drugs can also be taken up by the retina via receptor-mediated endocytosis by Müller cells, which may play an important role in retinal pharmacokinetics. Lastly, endogenous proteases might also play a role in drug degradation.
Traditional compartmental pharmacokinetic models assume a homogenous drug concentration, but this is likely not realistic given the heterogeneous nature of the vitreous body at different ages and in different diseases. Finite element modeling (FEM)-based models build upon the traditional compartmental model by using three-dimensional models with thousands of microscopic compartments that are associated with specific tissues and incorporate tissue-specific physiologic data. , These newer models have afforded helpful insights into vitreous pharmacokinetics. Studies by Zhang et al. have shown that significant mixing occurs immediately after injection, with intravitreal drugs occupying 2 to 10 times their initial injected volume. The exact degree of mixing likely varies depending on viscosity of the drug and vitreous viscosity, which may vary based on factors such as age, myopia, diabetes, and prior ocular procedures. Peak intravitreal drug concentrations vary depending on injection factors such as needle position, needle gauge, and speed of injection. , However, the long-term drug concentration profiles are apparently less impacted by initial distribution and depend primarily on the injected dose. , In these studies, clearance of intravitreal drugs depended on molecular size, and retinal delivery of drugs depended on RPE and ILM permeability, consistent with the aforementioned.
Embryology and development
The vitreous body develops through two embryologic stages called the primary and secondary vitreous. Older literature speaks of a tertiary vitreous, but this is actually the zonular system of the lens composed of elastin and not vitreous collagen. A significant limitation in our understanding of vitreous embryology is the lack of a clear understanding about which cells synthesize various components of the vitreous body and at what time points in development.
Primary vitreous
In early stages, the optic cup is mainly occupied by the lens vesicle. As the cup grows, it is filled by fibrillar material, presumably secreted by the cells of the embryonic retina, but possibly the ciliary epithelium. Later, with penetration of the hyaloid artery, more fibrillar material apparently originating from the cells of the walls of the embryonic vasculature and adjacent cells (hyalocytes?) fills the space between the developing lens and retina.
Inner limiting membrane and Bruch’s membrane continuity
During invagination of the optic vesicle the primary vitreous forms between the lens and the ILM of the retina. It is noteworthy that the ILM is continuous with Bruch’s membrane ( Fig. 6.14 ), demonstrating a common embryologic origin with analogous molecular composition and structure, suggesting important similarities later in life. ,

Secondary vitreous
The secondary vitreous appears at the end of the 6th week, associated with increasing size of the vitreous body and regression of the hyaloid vasculature. Primordial cells of the primary vitreous differentiate into hyalocytes and fibroblasts in the secondary vitreous.
Regression of hyaloid vascular system
A unique aspect of vitreous development is growth ( Fig. 6.15 ) and regression ( Fig. 6.16 ) of the fetal hyaloid vasculature during the first two trimesters of human embryogenesis. Vitreous vascularization begins with the hyaloid artery entering the nascent eye through the optic cup. By 10 weeks’ gestation (WG), the hyaloid system is well established, branching to form the vessels of the vasa hyaloidea propria, which anastomose to a dense capillary network surrounding the lens, the tunica vasculosa lentis. Maximal development of the hyaloid vasculature is reached by 12 to 13 WG. Regression of the fetal hyaloid vasculature results from downregulation of cell metabolism driven by reactive oxygen species-induced apoptosis, and concurrent upregulation of connective tissue synthesis in the extracellular space ( Fig. 6.16 ).


In a proteomics study of vitreous bodies from 17 human embryos aged 14 to 20 WG, 1217 proteins were detected, of which 768 proteins were not previously identified in the literature. After quantile normalization and variance filtering, 206 unique proteins were analyzed, finding that the peptide counts of 37 proteins changed significantly from 14 to 20 WG: 9 decreased while 28 increased. Among those with decreased expression were cofilin-1, which has been shown to promote/mediate angiogenesis; peroxiredoxin, which may play an antioxidant protective role; and the glycolytic enzyme enolase. Notable in the group that increased were pigment epithelium-derived factor, which has been shown to be important in antiangiogenesis, and cytochrome C, a known mediator of developmental apoptosis. Immunohistochemistry localized proteins to the hyaloid artery, vasa hyaloidea propria, and tunica vasculosa lentis ( Fig. 6.17 ), and changes were noted during this period of vitreous embryogenesis. For example, clusterin and cadherin were not detected in 14 WG vitreous but did appear in the hyaloid vasculature by 18 WG. This is consistent with the increase in peptide counts detected in the proteomic analyses.

Fetal hyaloid vessels show clear signs of regression by 13 to 15 WG, beginning in the vasa hyaloidea propria followed by the tunica vasculosa lentis, and then the pupillary membrane. , This regression may be induced by decreased levels of VEGF along with autophagy and apoptosis. Blood flow in the hyaloid artery ceases at the 240-mm stage (7 months’ gestation). Regression of the vessel begins with glycogen and lipid deposition in the endothelial cells and pericytes of the hyaloid vessels. Endothelial cell processes fill the lumen, and macrophages form a plug that occludes the vessel. The cells in the vessel wall then undergo necrosis and are phagocytosed by hyalocytes. Dying endothelial cells project into the capillary lumen and interfere with blood flow, stimulating synchronous apoptosis of downstream endothelial cells (“secondary apoptosis”) and ultimately obliteration of the vessel. Vessel atrophy begins posteriorly, leading to the prevailing concept that posterior development of the avascular secondary vitreous induces regression of the vascular primary vitreous in a posterior to anterior direction through displacement and compression. However, this prevailing concept has recently been challenged by Bu et al., who suggested that interactive remodeling of the developing vitreous may be the case, rather than displacement by the secondary vitreous.
After fetal hyaloid vessel regression, the main hyaloid artery leaves in its wake a tubular structure surrounded by the secondary vitreous. This courses from the retrolental space of Berger to the optic nerve (area of Martegiani) and is called Cloquet’s canal ( Fig. 6.1 , right; Fig. 6.18 ). The studies of Jan Worst, who defined this as one of the cisterns within the vitreous body, suggest that this is a liquid-filled canal, which is likely to be true. Thus, the (intentional or incidental) injection of drugs into this structure might result in preferential transport to the optic disc, which might be beneficial or deleterious, depending upon the circumstances.

Growth
During childhood the vitreous body undergoes significant growth. The length in the newborn eye is approximately 10.5 mm, and by the age of 13 years, axial length increases to 16.1 mm in the male. The average adult vitreous is 16.5 mm long. , , Recent studies using computed tomography (CT) imaging to measure the volume of the human vitreous “chamber” (not the vitreous body) found a volume of 4.65 ± 0.4 mm 3 for women and 4.97 ± 0.5 mm 3 for men. There was a significant correlation between vitreous chamber volume and age, as well as axial length. Although this study did improve upon previous ones by excluding contributions of the retina and choroid, the state of the actual vitreous body was not assessed; specifically, there was no consideration given to the presence or absence of posterior vitreous detachment and how that influences volumetric measurements of the vitreous body itself.
Developmental anomalies
Developmental anomalies can arise from inherited genetic abnormalities, irregular vitreous development, or abnormal regression of fetal hyaloid vessels, resulting in persistent fetal vasculature (PFV) syndrome, which accounts for 4.8% of blindness in infants. Abnormal fetal vasculature regression occurs in 95% of premature infants and 3% of full-term infants, owing to multiple causes. , The majority of cases are sporadic, but some are inherited. Studies have found that the germline deletion of Bim, a proapoptotic factor, results in persistent hyaloid vasculature, increased retinal vascular density, and stunted retinal vessel regression in response to hyperoxia. Astrocytes have been shown to ensheath persistent hyaloid arteries in cases of PFV, suggesting a role in the persistence of these fetal vitreous vessels. Many other genes, growth factors, and molecular pathways have been implicated, including the Wnt signaling pathway, VEGF, and apoptotic factors such as p53 and angiopoietin 2. Incomplete regression of the hyaloid vasculature might underlie the presence of branching linear structures within the vitreous body that sometimes cause floaters and vision degrading myodesopsia (see section: Symptomatic posterior vitreous detachment). Indeed, given that both incomplete embryonic vessel regression and myopia are developmental abnormalities, there may be shared pathogenetic aspects that underlie myopic vitreopathy, a leading cause of vision degrading myodesospsia. ,
Vitreous aging
The vitreous body undergoes considerable physiologic changes during life that have great impacts on its structure and function. The fundamental aging change is fibrous liquefaction of the gel structure. This process begins early in life and progresses until about 70% of patients develop advanced, symptomatic, fibrous liquefaction by their eighth decade of life. ( Fig. 6.19 )
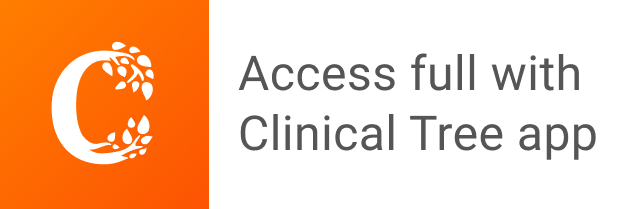