The bulk of the retina’s computing hardware is located in the inner retina. Here, dozens of neural circuits allow the retina to detect subtle changes in brightness, contrast, color, and other, more complex visual features, including oriented edges, motion direction, and object motion. All of these circuits adapt seamlessly over the enormous range of light levels and contrasts in natural scenes (reviewed in refs ). In this chapter, our focus is on the mouse, which is currently one of the best-studied nonprimate mammalian model systems in vision research. As evolutionary pressures drive visual systems to adapt to a species’ ecological niche (reviewed in ref ), mice, like other species, feature retinal specializations that are not found in, for example, primates, and we point out where such differences occur. However, given that the fundamental bauplan of the vertebrate retina is surprisingly well conserved, most of the computational principles and neural mechanisms we describe in this chapter apply to other species ( Fig. 23.1 ).

The players in the inner retina
At first glance, the organization of the retina may seem simple ( Fig. 23.1A ). Along an excitatory “vertical” pathway, photoreceptor signals are relayed by the bipolar cells (BCs) from the outer retina to the retinal ganglion cells (RGCs) in the inner retina (reviewed in ref ). The RGCs are the eye’s output neurons; their axons form the optic nerve, which projects to tens of visual centers, , and are the sole conduits for all the visual information required by the rest of the brain. Synaptic transmission along this vertical pathway is modulated by laterally organized interneurons—at the photoreceptor-BC synapse by horizontal cells (see Chapter 22 ), and at the BC-RGC synapse by amacrine cells (ACs) (reviewed in refs , ). This deceptively simple scheme is complicated by a rich diversity of cell types that form the many parallel circuits. In mouse, for example, the three different photoreceptor types (rods, M-cones, and S-cones; see Chapter 19 ) are contacted by 14 different BCs, which distribute their signals to approximately 45 different RGC types, each carrying a distinct representation of the visual world (reviewed in refs ). Moreover, the inner retina of the mouse features at least 63 types of AC, highlighting the computational importance of inner retinal circuits.
Bipolar cells
BCs are the sole forward connection between the outer and inner retina. Like photoreceptors, they employ ribbon synapses to release glutamate and hence provide the excitatory drive to the inner retinal circuits (reviewed in ref ). Among the retinal cell classes with more than a few types, BCs are probably the best understood class in terms of type classification and function. In the mouse, anatomical, , , genetic, and functional data converge on a set of 14 distinct types, plus an unusual dendrite-lacking BC type.
The dendrites of BCs receive synaptic input from photoreceptor axon terminals in the outer plexiform layer. One traditionally distinguishes between a single type of rod bipolar cell (RBC) and several types of cone bipolar cells (CBCs), depending on the photoreceptor subclass contacted. However, this is not clear-cut, as RBCs may also contact cones—in mice, more than half of them do —and some types of OFF CBCs additionally contact rods. , , Although the signaling in RBCs and CBCs is quite distinct, these pathways are intimately intertwined (see further). In addition, some CBC types make selective contacts with a single spectral type of cone , , and hence carry chromatic signals (see Chapters 22 and 34 ).
In addition to the photoreceptor types contacted, the responses of the different BC types are shaped by a number of mechanisms. For instance, the polarity of a BC’s light response depends primarily on the kind of postsynaptic glutamate receptor (GluR) it expresses. As photoreceptors release glutamate in darkness (see Chapter 19 ), the presence of sign-inverting metabotropic GluRs renders BCs responsive to light increments (ON BCs), whereas expression of sign-preserving ionotropic GluRs result in responses to light decrements (OFF BCs). Moreover, the specific GluR type expressed by OFF BCs contributes to tuning the temporal profile of the cells’ light responses. In general, OFF CBCs respond more quickly to luminance changes and adapt more rapidly to changes in contrast than their ON CBC counterparts. Finally, BC output is critically shaped by synaptic interactions with ACs at the BC axon terminal in the inner plexiform layer (IPL), as will be discussed in detail next.
Amacrine cells
With more than 60 types as suggested by mouse transcriptomic data, ACs are the most diverse class of retinal neurons (reviewed in refs , ). At the same time, the function of fewer than a dozen of them is currently well understood, making them the greatest challenge in fully understanding retinal information processing (reviewed in ref ).
ACs are roughly divided into narrow-field cells with arbor diameters of around 100 µm and less, and wide-field cells, whose arbors can span across several millimeters. Amacrine cells receive synaptic input from BCs and other ACs and provide synaptic output to BCs, ACs, and RGCs. Despite their name (amacrine ~ axonless), not all ACs lack an axon, especially the largest ones, which in fact have multiple axons (polyaxonal ACs , ). Nevertheless, most ACs use their dendrites for both receiving synaptic input and making output synapses (dendritic processing, see further; reviewed in refs , ). Because of this dual function, we will refer to the processes of axon-lacking ACs as neurites.
Many ACs are “bilingual”—they release two types of signaling molecules, like many interneurons in the brain (reviewed in ref ). The first one that is released is GABA or glycine, thus a fast, inhibitory transmitter. In mammals, most narrow-field ACs are glycinergic, whereas most wide-field ACs are GABAergic. The second one can be a transmitter but is typically a slower-acting neuromodulator (reviewed in ref ). How and where these transmitters are released depends on the AC type. For instance, VGluT3 (vesicular glutamate transporter type 3) ACs, which are involved in motion detection, release glycine and glutamate from synapses in distinct partitions of their neuritic arbors. In contrast, dopaminergic ACs, which play a key role in adjusting the retina’s light sensitivity, co-release GABA and dopamine, with the ratio of these molecules varying between vesicles and synaptic location. Starburst ACs, which are central to the circuit that computes direction selectivity (reviewed in ref ), corelease GABA and acetylcholine likely from different vesicle pools, , with GABA relaying local inhibition and acetylcholine perhaps acting on several spatial scales. ,
Retinal ganglion cells
Finally, RGCs pool many synaptic inputs from BCs and ACs impinging on their dendritic arbor and convert the resulting signal into a spike train in the optic nerve. A few RGC types strongly express the visual pigment melanopsin, which renders these cells intrinsically photosensitive (ipRGCs) and enables them to provide critical input for photoentrainment of the biologic clock , (see Chapter 26 ). Whether these strongly melanopsin-positive cells also contribute to image-forming vision is still a matter of debate (reviewed in ref ). In mice, evidence from functional, anatomical, and genetic studies jointly point at a total set of at least 45 types of RGCs (see also http://rgctypes.org/ ).
Each type of RGC can be thought of as the end point of a retinal circuit that extracts and encodes a representation of distinct visual features (reviewed in refs , ). The RGCs with the smallest dendritic arbors encode the finest spatial details; here, at the extreme, are the midget RGCs in the primate fovea (reviewed in refs , ), which make private-line connections between a single cone (via a single BC) and the brain (with receptive field centers of ~1/60 degrees visual angle ). In mice, which lack a fovea and midget cells, the representations with the highest spatial resolution (~3 degrees visual angle) are likely implemented by the “high-definition” cells (HD RGCs ). Among the largest RGCs are the alpha cells, a group of RGC types that differ in response polarity and transience. As they are strongly driven by RBCs and pool over a large retinal area, they remain responsive even at the limit of vision when photons are sparse. , Notably, at least one of these alpha cells, the transient OFF alpha RGC, performs other functions at higher light levels. It responds to approaching dark objects, potentially warning the animal of approaching predators, and it can signal image recurrence associated with saccadic eye movements. Other visual features encoded by different RGCs (some of which are detailed in the next section) include motion direction, which can support image stabilization, object motion, contrast, , color, and orientation.
The playing field
The playing field is the IPL, where the axon terminals of BCs, the neurites of ACs, and the dendrites of RGCs interconnect. The IPL is organized in a laminar fashion ( Fig. 23.1 ), for example, OFF responses dominate the distal portion of the IPL (toward the AC somata), ON responses the proximal portion (toward the RGC somata), and the fastest, most transient responses are clustered roughly toward the IPL center. This organization is implemented by different BC and AC types stratifying at distinct IPL depths, where they provide specific combinations of functional output, which, in turn, can be picked up by an RGC that selectively targets those IPL depth(s) with its dendrites. The key computational units of the IPL are (1) the BC axon terminal, (2) the AC dendrites, and (3) the RGC dendritic arbors, all of which can perform a wide range of complex nonlinear computations using a similarly broad spectrum of cellular mechanisms.
Bipolar cell axon terminals
The terminals of BCs receive massive feedforward and/or feedback inhibition often from multiple AC types, critically shaping a BC’s direct photoreceptor input signal (see Chapter 22 ) and, hence, its glutamatergic output (reviewed in ref , see also Box 23.1 ). The GABAergic AC input tunes the temporal properties of the BC output depending on the postsynaptic receptor types (e.g., GABAA vs. GABAC ). Moreover, in concert with glycinergic inhibition, GABAergic AC input forms the BC’s antagonistic center-surround receptive field. , In fact, AC input can drive a BC’s response alone, which can be demonstrated by presenting an annular stimulus in a BC’s antagonistic receptive field (RF) surround while leaving out its RF center (i.e., the direct photoreceptor input ). Thus, BC output always also reflects AC activity.
Glutamate release from BCs is further modulated by several mechanisms. For example, the biophysical properties of the ribbon synapse render BC output sensitive to the recent history of its activity. Retinal contrast adaptation, for instance, partially relies on the availability and priming state of synaptic vesicles at the BC ribbon synapse. Next, the ion channel complement present in axon and axon terminal varies between BC types, enabling some BCs to generate spikes (reviewed in ref ), which further extends their temporal signaling capabilities. , Finally, many of the neuromodulators released by ACs can fine-tune BC output. It has been shown, for example, that dopamine, neuropeptide Y, and endocannabinoids , modulate voltage-gated Ca 2+ and/or K + channels in BC terminals ( Box 23.1 ).
Vertical pathway, lateral interactions, and computational units. The transformation of the photoreceptor signal by bipolar cells (BCs) is often approximated in simple linear-nonlinear (LN) models. Here, the sum of all linear operations within the BC is combined into a single representation (impulse response). Convolution of this impulse response with a stimulus, followed by the passing of the result through a “static nonlinearity,” yields a prediction of the cell’s output to that stimulus. In the figure, we illustrate how elements of such LN models (center) may be mapped onto a BC’s dendrite, soma, axon, and axon terminals; the respective synaptic sites in the circuit are depicted to the sides ( left : input from photoreceptors and output to retinal ganglion cells [RGCs]; right: lateral interactions with horizontal cells (HCs) and amacrine cells (ACs). Note that colors mark linear operations (black) , nonlinear operations (red) , and time-dependent processes (blue) .
In step ( 1 ), the synaptic input the BC receives at its dendrites is temporally filtered (i.e., by the neurotransmitter receptors, the passive soma + axon).
In step ( 2 ), the signal from the axon is integrated at the axon terminals with local processes, including AC input, electrical gap-junctional coupling, and active channels (here approximated by a single LN element). Step ( 3 ) accounts for the potential presence of spiking activity. The result of step ( 2 ) (together with step ( 3 ), if included) is the activation of voltage-gated Ca 2+ channels in the terminals. In step ( 4 ), the Ca 2+ entering the terminal is subject to buffering, diffusion, and extrusion by pumps. As a result, bulk Ca 2+ within the terminal imposes another low-pass filter onto BC activity before triggering the release of glutamate-filled vesicles. Depending on the vesicle-supply state of the synaptic ribbon, release can be highly nonlinear or roughly linear. Notably, BC synaptic release not only affects RGCs, but can also elicit input from ACs (step [ 5 ]), which may act through direct (reciprocal) or indirect (via a series of ACs) neurotransmission, and/or through neuromodulators.

Amacrine cell dendritic compartments
The dendritic arbor of ACs is often functionally partitioned, for example, through a differential distribution of input and output synapses. This partitioning dramatically varies between AC types and does not necessarily correlate with arbor size. For instance, A17 cells, which belong to the wide-field ACs, feature more than 100 regularly spaced varicosities on their neurites with each varicosity providing independent inhibitory feedback to a single RBC axon terminal. , In contrast, in the similar-sized starburst ACs, each primary neurite is roughly divided into a proximal input and distal output zone, implementing a “detector” for motion direction. , Narrow-field ACs such as the A2 and the A8 often act as vertical “conducts” by relaying signals between different depths of the IPL.
Dendritic and axonal integration in retinal ganglion cells
The dendrites of RGCs integrate excitatory and inhibitory synaptic input from distinct combinations of BCs and ACs. How the RGC’s output signal is computed from this input depends on morphologic features, such as branching pattern, dendritic thickness, and segment length, as well as on the complement and distribution of ion channels, including not only voltage-gated Na + , K + , and Ca 2+ channels but also Ca 2+ -activated K + channels or hyperpolarization-activated cyclic nucleotide–gated (HCN) channels. These properties vary strongly between RGC types and impact dendritic integration. For instance, small-diameter dendritic segments with high axial resistance, on one hand, can result in shorter propagation distances of dendritic signals and, hence, more independent arbor regions. , On the other hand, expression of active Na + channels along the dendrite may enhance signal propagation and enable backpropagation of somatic spikes. Still, predicting how specific combinations of dendritic geometry and active channel densities affect dendritic integration is challenging. The final step in tuning a RGC’s output is the axon hillock, where cell type–specific combinations of active channels convert the voltage signal into a train of action potentials. Differences in the number and type of Na + channels at the axon hillock can exert important influences on spike generation. , , Taken together, RGCs themselves can perform nonlinear computations and therefore contribute to the functional properties of “their” information channel to higher visual centers.
Example circuits
The players of the inner retina can come together in countless configurations to support different visual computations, and many of these retinal circuits remain to be discovered. In the following sections, we describe five retinal circuits for which we have some clarity about which players are essential and the way in which they work together to achieve a particular computation. More details and additional circuits can be found.
Color polarity switching
Color vision (see Chapter 34 ) starts in the retina. Here, color-opponent circuits begin with BCs that sample selectively from spectrally different cone types and culminate in RGCs, the response polarity of which depends on wavelength (e.g., blue ON-yellow OFF ). The trichromatic (blue, green, red) visual system in humans is a relatively recent adaptation in primate evolution, resulting from a gene duplication that created separate green (medium wavelength, M) and red (long-wavelength, L) opsins, although trichromatic and tetrachromatic vision are evolutionarily ancient in nonmammals. Thus, human color vision is built from the ancient blue-yellow (short-wavelength, S, vs. M/L) opponent circuit and the separate, more recent green-red (M vs. L) circuit.
Most nonprimate mammals, such as mice, rabbits, and ground squirrels, have only S- and M-cone opsins, and they serve as models for human S vs. M color-opponent circuits. Whether color-opponent perception requires both RGC response polarities (S ON /M OFF and S OFF /M ON ) remains controversial ; nonetheless, different RGC types with each color opponent S/M polarity have been found in ground squirrels, rabbits, and nonhuman primates. S ON responses in RGCs have a straightforward anatomical substrate in S-cone–selective ON CBCs, which are well conserved across all of these species, as well as mice. , , , S OFF responses, however, are more difficult to explain, because there does not appear to be an S-cone–selective OFF CBC in mice, ground squirrels, or primates, and its existence in rabbits remains unresolved.
The solution to the question of how to build an S OFF RGC is to invert the S ON signal with an AC. A landmark study identified a narrow-field S ON /M OFF AC in the ground squirrel retina that is likely to receive its excitatory input from S ON CBCs and provide glycinergic inhibition to S OFF /M ON RGCs. Thus the RGC is hyperpolarized by this inhibitory input for S ON or M OFF and depolarized by disinhibition for S OFF or M ON . Pharmacology experiments suggested that a similar circuit exists in the rabbit, but the respective AC has not yet been found ; circuit mechanisms of the S OFF /M ON RGCs in primate retina have not been investigated. Similar to the role of other narrow-field ACs in “crossover” inhibition between ON and OFF BC pathways, this color-inversion circuit represents a “vertical” computation that acts within a narrow region of the visual field to process information between BC channels.
Circuits for night vision
Rod photoreceptors enable night (scotopic) vision. Their phototransduction cascade (see Chapter 18 ) and synaptic release kinetics are optimized to catch and signal when photons are scarce. At light levels below around 100 P*s −1 (photoisomerization per second; mesopic range) per rod, the retina switches from cone- to rod-dominated signaling. There is, however, evidence that rods remain light sensitive and contribute to daylight (photopic) vision.
Rod signals can reach the RGCs via different pathways. In the primary rod pathway ( Fig. 23.2A ), the aforementioned RBCs rarely connect to RGCs directly; instead, this pathway feeds into the CBC circuits. This piggyback arrangement allows sharing of the same feature-extracting hardware in the inner retina during night and day. Specifically, RBCs possess their “own” AC, the A2, which relays RBC signals to the axon terminals of OFF CBCs via inhibitory glycinergic synapses in the distal IPL, and of ON CBCs via gap junctions (electrical synapses) in the proximal IPL (reviewed in ref ). By inverting the RBC signal in the distal but not the proximal IPL, the A2 connectivity ensures that signals reach OFF and ON CBCs, respectively, with the proper polarity. Interestingly, at daytime, A2 ACs are repurposed to serve a circuit that detects approaching dark shapes, , illustrating how intimately retinal circuits are entwined. In addition to the RBC-A2 mediated pathway, rod signals can also reach CBCs via electrical rod-cone coupling ( Fig. 23.2A ; ); this secondary rod pathway has been shown to exist in many species, including primate and mice. Finally, as some OFF CBC types contact rods in addition to cones, rod signals can enter the OFF CBC circuits directly, forming a tertiary rod pathway. , Recently, a further pathway has been discovered—some RGC types may receive direct glycinergic A2 input to their somata.

In addition to intrinsic rod properties, it is the tremendous convergence of up to thousands of rods per RGC ( Fig. 23.2B ) that yields high amplification and reduction of noise in the primary rod pathway, allowing it to signal at starlight. , In addition, rod signals are differentially processed by distinct retinal circuits, making visually guided behavior at the limit of vision possible. For instance, some RGC channels are extremely light sensitive but noisy (high false-positive rate), whereas others signal more reliably at the cost of sensitivity (high false-negative rate). , ,
Object motion sensitivity
Motion is ubiquitous in most visual environments, and different types of motion, like those of predators and prey, carry special behavioral significance. An animals’ own movements also cause motion on its retina, hence it is critical to distinguish self-motion from that of external objects. The computation that identifies object motion amidst a background of global motion begins in the retina, where a set of object motion–sensitive (OMS) RGC circuits ( Fig. 23.3A ) effectively subtract global motion and respond selectively to moving objects Fig. 23.3B ).

The OMS circuit was first identified in the salamander retina. It relies on wide-field ACs that capture signals from the receptive field (RF) surround and provide inhibitory input to BC axon terminals in the RF center, thereby canceling BC excitation for global motion covering both the RF center and surround. Despite its name, the OMS circuit does not rely on an explicit representation of motion in the RF center or surround. Instead, it uses nonlinear BC subunits to respond to any spatiotemporal contrast. Thus, the requirement for global motion cancellation is that contrast edges in the RF center and surround move at the same time regardless of their size, contrast, direction, or other details.
Without advanced genetic tools, it has been difficult to associate specific retinal neurons with OMS circuits in salamander or rabbit retinas, so researchers have turned to the OMS RGCs in mice. The first piece of evidence about the components of the mouse OMS circuit was that it relied on spiking ACs because it was blocked by the voltage-gated Na + channel antagonist Tetrodotoxin (TTX). Initially, OMS was identified in what was thought to be a single, genetically identified RGC type, but it was later shown to be present in at least several other RGC types, depending on the kind of stimuli used. A GABAergic wide-field AC type called TH (tyrosine hydroxylase) 2 was identified as a component of one OMS circuit in the mouse. However, unlike the spiking ACs implicated in the original study, TH2 cells do not fire action potentials and are unaffected by TTX. Excitation to OMS RGCs may be provided by both BCs and a glutamatergic AC called VG3. , Because the OMS circuit is based on circuit motifs that are common in the retina ( Fig. 23.3A ), and nonlinear subunits in the RF center and surround, it is possible that variations of this computation occur in parallel, upstream of multiple RGC types. It is conceivable that OMS is multiplexed with other retinal computations at the level of RGCs. Recent work in salamander has identified RGCs that are both OMS and direction selective (DS).
Direction selectivity
Another important property of visual motion is its direction, and therefore it is not surprising that DS neurons are found across species and at different levels of the visual system, including the retina (reviewed in refs , ) ( Fig. 23.4 ). Nearly 60 years ago, Barlow and Levick studied the rabbit retina and found DS RGCs—so-called DSGCs—that spiked vigorously when an object moved in a specific direction across the cell’s RF ( Fig. 23.4A2 ). Since then, many researchers have investigated DS circuits and mechanisms, particularly in rabbits and mice, making it one of the most studied and best understood retinal computations (reviewed in refs ).

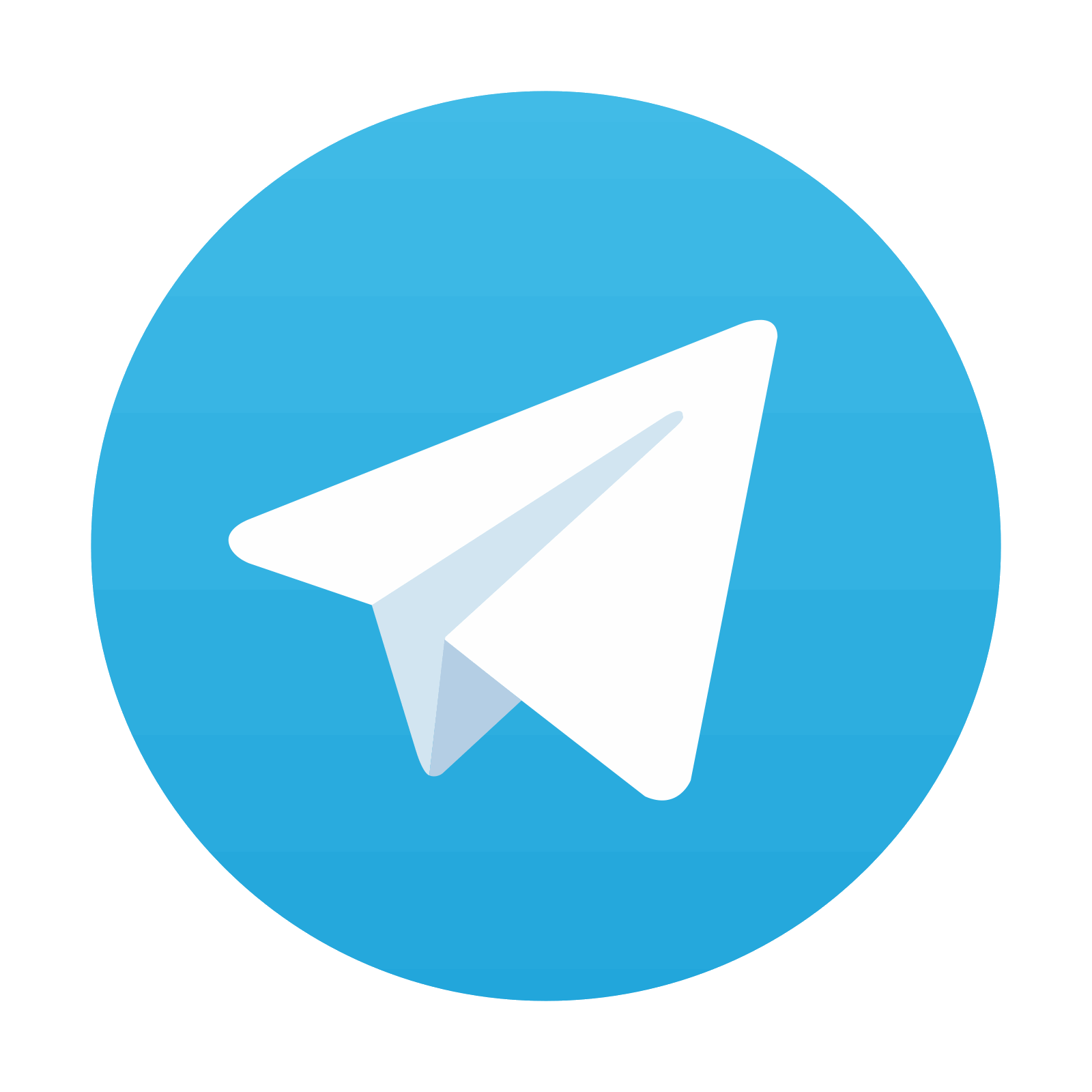
Stay updated, free articles. Join our Telegram channel
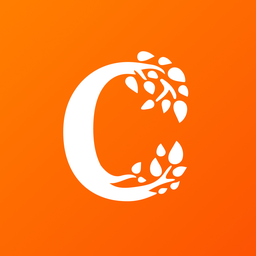
Full access? Get Clinical Tree
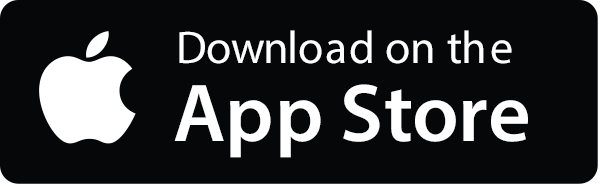
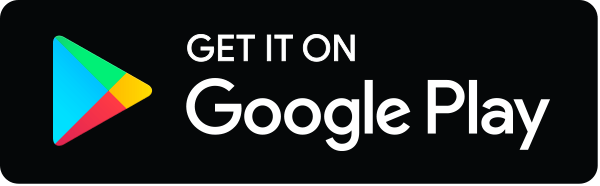
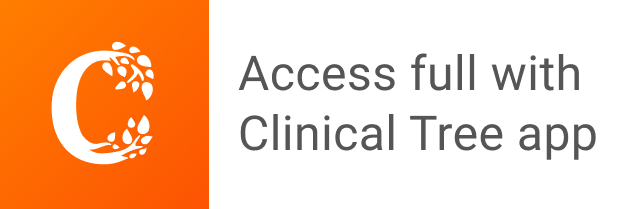