Introduction
The vitreous body makes up approximately 80% of the volume of the eye and thus is the largest single structure of the eye ( Fig. 6.1 ). In the anterior segment of the eye, it is delineated by and adjoins the ciliary body, the zonules, and the lens. In the posterior segment of the eye, the vitreous body is delineated by and adjoins the retina.

The vitreous body has many normal physiological functions. This chapter focuses on the most important physiologic relationships, especially those that have a close clinical correlation. As background for the understanding of the physiology and the pathophysiology of the vitreous body, we focus on the main features of the anatomy, biochemistry, and biophysics.
The investigation of the vitreous body and its structure and function is hampered by two fundamental difficulties. Firstly any attempts to define vitreous morphology are in fact attempts to visualize a tissue, which by design is intended to be invisible. Secondly the various techniques that have previously been employed to define the structure of the vitreous body are combined with artifacts that make interpretations difficult in terms of the true in vivo physiological situation.
Anatomy
Embryology
Structural considerations of embryology
In the early stages the optic cup is mainly occupied by the lens vesicle. As the cup grows the space formed is filled by a system of fibrillar material, presumably secreted by the cells of the embryonic retina. Later, with the penetration of the hyaloid artery, more fibrillar material apparently originating from the cells of the wall of the artery and other vessels contribute to filling the space. The combined mass is known as primary vitreous .
The secondary vitreous develops later, appearing at the end of the sixth week, and is associated with the increasing size of the vitreous cavity and the regression of the hyaloid vascular system. The main hyaloid artery remains for some time, but it eventually disappears and leaves in its place a tube of primary vitreous surrounded by the secondary vitreous, running from the retrolental space to the optic nerve (area of Martegiani). The tube is called Cloquet’s canal ( Fig. 6.1 ); this is not a liquid-filled canal, but simply a portion of differentiated gel devoid of collagen fibrils.
The term tertiary vitreous is related to the fibrillary material, which develops as the suspensor fibrils, the zonules, of the lens. During childhood the vitreous undergoes significant growth. The length of the vitreous body in the newborn eye is approximately 10.5 mm, and by the age of 13 years, the actual length of the vitreous increases to 16.1 mm in the male. In the absence of refractive changes, the mean adult vitreous is 16.5 mm.
Molecular and cellular considerations of embryology
The two main components of the vitreous, collagen and hyaluronic acid, are produced in the primary and secondary vitreous. In the primary vitreous, however, there is initial production of substances other than hyaluronic acid, such as galactosaminoglycans; later hyaluronic acid becomes the predominant constituent.
The primary vitreous contains cells which in the secondary vitreous differentiate as hyalocytes and fibroblasts. The hyalocytes are believed to be involved in the production of glycosaminoglycans, especially hyaluronic acid, a non-sulfated glycosaminoglycan.
Although the function of the fibroblasts is not known exactly, they are probably involved in the formation of collagen. The retina may also be a source of collagen synthesis. The hyalocytes are found in the vitreous cortex, approximately 30 µm from the internal limiting membrane (ILM), with the highest density near the vitreous base and the posterior pole.
Anatomy of the mature vitreous body
The mature vitreous body is a transparent gel which occupies the vitreous cavity. It has an almost spherical appearance, except for the anterior part, which is concave, corresponding to the presence of the crystallin lens. The vitreous body is a transparent gel; however, it is not completely homogeneous ( Fig. 6.2 ). The outermost part of the vitreous, called the cortex, is divided into an anterior cortex and a posterior cortex, the latter being approximately 100 µm thick ( Fig. 6.3 ). The cortex is also called the anterior and the posterior hyaloid . The cortex consists of densely packed collagen fibrils ( Fig. 6.4 ). The vitreous base (see Fig. 6.1 ) is a three-dimensional zone. It extends approximately from 2 mm anterior to the ora serrate to 3 mm posterior to the ora serrata, and it is several millimeters thick. The collagen fibrils are especially densely packed in this region.



The vitreoretinal interface
The vitreoretinal interface can be defined from electron microscopy as the outer part of the vitreous cortex (posterior hyaloid), including anchoring fibrils of the vitreous body and the ILM of the retina ( Fig. 6.5 ). The ILM is a retinal structure between 1 and 3 µm thick, consisting mainly of type IV collagen and proteoglycans. It contains several layers and can be considered the basal lamina of the Müller cells, the foot processes of which are in close contact with the membrane.

The vitreous cortex is firmly attached to the ILM in the vitreous base region, around the optic disc (Weiss ring), at the vessels, and in the area surrounding the foveola at a diameter of 500 µm. Under normal conditions, the connection between the fibrils of vitreous cortex and the ILM is looser than in the rest of the vitreoretinal interface. The adhesion is strong in young individuals, and dissection of the retina from the vitreous often leaves ILM tissue adherent to the vitreous cortex. Under pathologic conditions, the tight connections between the vitreous cortex and the ILM play an important role, as is discussed later in this chapter.
Ultrastructural, biochemical, and biophysical aspects
Ultrastructural and biochemical aspects
The vitreous contains more than 99% water; the rest is composed of solids. The vitreous acts as a gel (i.e. an interconnected meshwork) that surrounds and stabilizes a large amount of water compared with the amount of solids. The gel structure of the vitreous results from the arrangement of long, thick, non-branching, collagen fibrils suspended in a network of hyaluronic acid, which stabilize the gel structure and the conformation of the collagen fibrils ( Figs 6.6 and 6.7 ).


In the human eye the major part of the glycosaminoglycan is hyaluronic acid, with a molecular weight of 3–4.5 × 10 6 . The volume of non-hydrated hyaluronic acid is 0.66 cm 3 /g, in contrast with the volume of the hydrated molecule, which is 2000–3000 cm 3 /g. The molecule forms into large, open coils, with the anionic sites spread apart. This arrangement of small-diameter fibers, separated by highly hydrated glycosaminoglycan chains, permits the transmission of light to the retina with minimal scattering. The collagen fibrils in the vitreous are thin, with diameters of approximately 10–20 nm. Collagen fibrils are mostly of collagen type II. They are composed of three identical α-chains, which form a triple helix. The helix is stabilized by hydrogen bonds between opposing residues in different chains. Collagen type IX is also present and may function as a bridge, linking type II collagen fibrils together. Collagen V/XI is integrated with collagen II in the collagen fibers. The collagen fibrils seem to interconnect with the hyaluronic acid, most likely via bridging glucoproteins. The viscoelastic properties of the vitreous gel are neither due to hyaluronic acid or collagen alone but to the combination of the two molecules.
Dissolved in the water of the vitreous gel are inorganic and organic substances as shown in Table 6.1 , where plasma values are given for comparison.
Organic substances | |||||||
---|---|---|---|---|---|---|---|
Ascorbate | Glucose | Lactate | |||||
Vitreous | 0.46 | 3.0 | 12.0 | ||||
Plasma | 0.04 | 5.7 | 10.3 |
* Human data from McNeil et al 1999.
According to Table 6.1 , it appears that gradients exist in both directions between vitreous and plasma. These gradients are a result of several mechanisms: presence of the blood–ocular barriers (i.e. active and passive passage across the barriers), metabolism in retina and ciliary body, and diffusion processes in the vitreous body ( Box 6.1 ).
- •
The concentration of salts and organic substances of the vitreous differ substantially from plasma due to the blood–aqueous and blood–retinal barrier
- •
Small molecules move through the vitreous gel by diffusion
- •
Vitreous fluorometry is useful for evaluation of the vitreal morphology, the fluorescein profile is an indicator of physiologic aging such as vitreous liquefaction
- •
The aging process leads to posterior vitreous detachment, easily visualized by optical coherence tomography (OCT)
- •
Vitreoretinal traction may lead to formation of a macular hole and the traction can be conducted through the retinal layers
- •
Vitreoretinal traction is also implicated in some cases of macular edema
- •
In diabetes, the high glucose speeds up metabolism before visible retinopathy
- •
Increased demand for oxygen and capillary closure leads to retinal ischemia and an increased production of VEGF
- •
Increased leakage through the blood–retinal barrier leads to macular edema
- •
VEGF inhibition and steroids decrease macular edema
The values in Table 6.1 represent mean values for the whole vitreous. The methods used to quantitate vitreous concentrations are difficult and may differ between studies in absolute numbers. However, regional differences within the vitreous have been measured for some substances. Figures 6.8 to 6.10 show the regional difference for glucose ( Fig. 6.8 ), lactate ( Fig. 6.9 ), and oxygen ( Fig. 6.10 ). The fall in vitreous oxygen tension towards the center, corresponding to the upper curve in Figure 6.10 , was also found by Sakaue and seems to result from an oxygen flux from the retina towards the vitreous corresponding to arterioles; the flux goes in the opposite direction corresponding to the venules (lower curve). Several studies have found an increase in preretinal oxygen after photocoagulation, indicating that the oxygen supply to the inner retina improves after destruction of the outer retina and a concomitant decrease in tissue metabolism and oxygen needs.



Biophysical aspects
The gel structure acts as a barrier against movement of solutes. Basically, substances may move by two different processes: diffusion or bulk flow. The diffusion process can be illustrated in humans by using fluorescein as a tracer substance for the biophysical behavior of the gel. The fluorescein concentration in the vitreous body can be estimated by vitreous fluorophotometry. After intravenous (IV) injection of fluorescein, a certain amount (in healthy humans only a very small amount) passes through the ocular barriers into the anterior chamber and into the vitreous body. The ILM, the vitreoretinal interface, and the vitreous cortex cannot be regarded as a diffusion restriction to smaller molecules ( Box 6.1 ). In the vitreous the distribution versus time occurs according to the diffusion properties of a particular molecule in the vitreous gel.
An analysis of the fluorescein concentration gradient in the posterior part of the vitreous can be made with the aid of a simplified mathematical model of the relationship between the vitreous body and the blood–retinal barrier, as shown in Figures 6.11–6.14 .




In the model the vitreous body is considered as a globe with an outer delineation corresponding to the blood–retinal barrier ( Fig. 6.11 ). Fluorescein passes the barrier passively with permeability P. Diffusion in the vitreous gel takes place with a diffusion coefficient D. The time-dependent plasma fluorescein concentration is given by Co(t) and the concentration in the vitreous body dependent on time (t) and distance (r) from the center of the eye is given by C(r,t).
The basic equations and the mathematical formalisms are as follows:
C ( r , t ) = ∫ 0 t C o ( t-s ) * F ( r , s ; a , D , P ) ds
F ( r , s ; a , D , P ) = aP r D * [ G ( a − r 2 D , s ; k ) − G ( a + r 2 D , s ; k ) ]
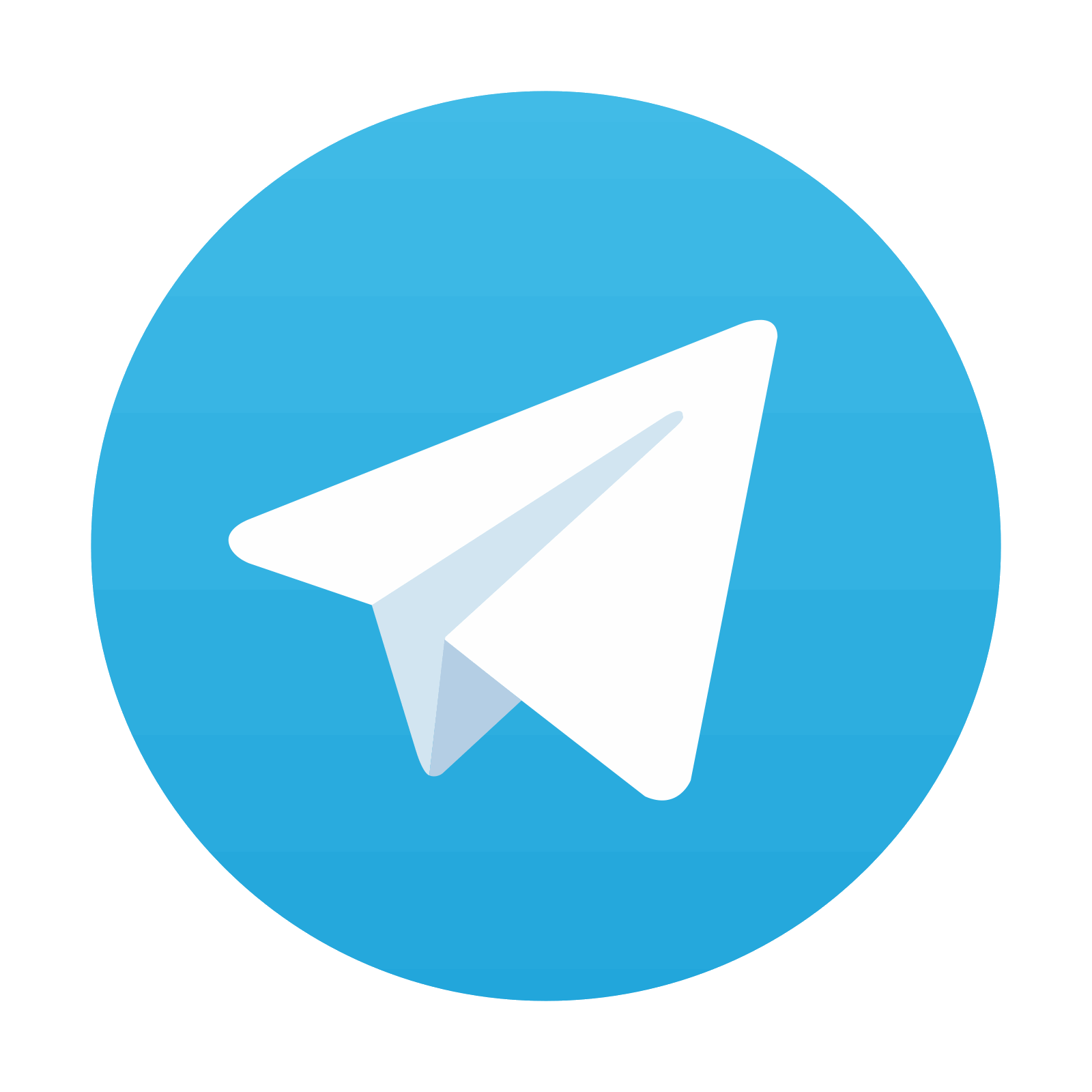
Stay updated, free articles. Join our Telegram channel
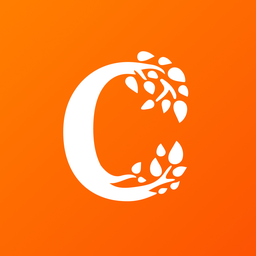
Full access? Get Clinical Tree
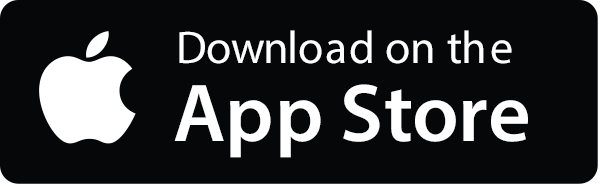
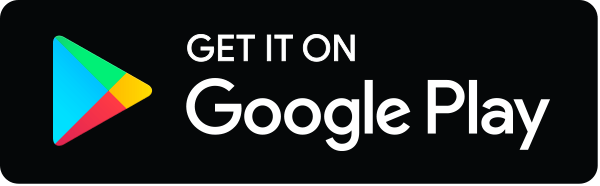