Introduction and historical background
Perimetry and visual field testing have been used as diagnostic procedures for evaluation of visual function for approximately 200 years. A historical review of these techniques may be found in several publications, with the onset of visual field testing occurring more than 2000 years ago. Particularly within recent times, a variety of new techniques for performing tests and interpreting results have been developed, but the primary method of assessing the visual field has been the detection of a small white stimulus superimposed on a uniform white background. Perimetry is used clinically to detect functional losses produced by pathology to the visual pathways, to provide differential diagnostic information concerning the location of a visual deficit, to monitor the status of the visual field over time to determine the efficacy of treatment and the stability of vision impairment, and to evaluate the condition of visual mechanisms subserving the peripheral visual fields. Recent enhancements of the test and analysis procedures have made it possible to automate the techniques, standardize the methodology, perform sophisticated statistical and mathematical evaluation of results, and allow rapid exchange of information among clinical sites.
In this chapter the psychophysical basis for visual field testing is discussed and an overview of the various forms of visual field testing that are available is provided. In addition, guidelines for interpretation of visual field results are presented, and determinations of the pattern, shape, and location of visual field losses are reported. A presentation of artifactual test results and how to avoid them is offered and methods of assessing progression and improvement of visual fields over time are evaluated. The chapter also provides a set of recommendations for clinical visual field testing, and introduces new methods of performing portable, accurate visual field testing in a variety of settings using tablets and virtual reality headsets. It is hoped that the reader will find this information helpful and be able to incorporate these techniques into their daily clinical regimen.
The psychophysical basis for perimetry and visual field testing
The most common form of perimetric testing is performed monocularly with the fellow eye occluded. The patient’s task is to detect a small white target stimulus briefly superimposed on a uniform background luminance at different visual field locations to determine the minimum increment of light needed to detect the stimulus (the differential light threshold or increment threshold), often referred to as Weber’s law (L/L = C, where L is the background luminance, ΔL is the increment of light, and C is a constant). Light sensitivity, the reciprocal of threshold, is presented either as the size and intensity of the stimulus that could be just noticeably detected at a particular visual field location for manual testing, or as a decibel (dB) value in automated perimetry, where 0 dB represents the maximum intensity that can be achieved by the device, and higher dB values indicating lower intensities on a log scale times 10 (inverse log scale). The dynamic range of an automated perimeter is typically from 0 to greater than 40 dB to cover the range of possible sensitivity thresholds for various stimulus sizes and eccentricities. There are a number of factors that affect the ability to detect a stimulus (visual field location, size, duration, color, flicker, motion, adaptation level, etc.) that will only partially be discussed in this chapter but have been reported elsewhere.
A standard background luminance of 31.5 apostilbs (10 candelas per meter squared [cd/m 2 ]) is typically employed to provide a photopic level of adaptation, maintain the consistency of Weber’s law, and provide the most stable testing conditions. Additionally, it is important to have a pupil diameter that is greater than 2 mm, an optimal refractive correction for the testing distance, and appropriate testing conditions (stimulus size and duration, interval between presentations, steady fixation, etc.). Under these conditions, the fovea is the most sensitive visual field location, thereby able to detect the smallest and dimmest stimulus. Sensitivity decreases systematically as one moves from the fovea toward greater visual field eccentricities, as indicated in Fig. 35.1 for a right eye, for which sensitivity is plotted three-dimensionally (x and y position coordinates and a z intensity coordinate) for the visual field. In view of this shape, this representation has often been called the “hill of vision” or the “island of vision in a sea of blindness.” The shape of the visual field will vary slightly when different stimulus sizes and durations are used. The dark hole in the hill of vision is the blind spot, where the optic nerve exits the eye to connect with the brain. It is a region of nonseeing because there are no photoreceptors or other retinal sensors present there. The location of the blind spot is approximately 15 degrees from the fovea on the temporal side of the visual field with about one-third of the blind spot above the horizontal meridian (2 degrees) and roughly two-thirds below the horizontal meridian (4 degrees). The overall size of the visual field is greater than 90 degrees temporally (away from the nose), 60 degrees nasally (toward the nose), 60 to 70 degrees superiorly, and 80 degrees inferiorly for a young, normal healthy eye.

In addition to testing conditions that can affect visual field sensitivity, properties of the visual pathways also influence differential light sensitivity. The ability to detect an increment of light is not all or nothing, but is probabilistic, which can result in variability of 2 to 3 dB in normal visual field areas and up to 10 or more dB in damaged visual field regions. This probabilistic aspect of detection sensitivity can be described by a frequency of seeing curve. Very dim stimuli are rarely or never seen, whereas high-intensity stimuli are nearly always detected. As a consequence, the frequency of seeing curve is S-shaped and spans a range of approximately 3 dB for locations near the center of the visual field and 4 to 5 dB for stimuli at more eccentric locations for a normal eye, and 10 dB or more in damaged visual field areas. The variability is usually different for individuals, depending on age, attentiveness, cognitive abilities, and related factors.
Another factor that can affect visual field sensitivity is damage produced by disease or other impairments to the visual pathways, which is its most important attribute as a clinical diagnostic test procedure. As indicated in later portions of this chapter, the pattern, shape, and location of visual field sensitivity losses can be helpful in identifying the location of visual pathway dysfunction and the potential disorders that may have produced this loss. In addition to overall sensitivity loss in damaged visual field areas, these compromised regions typically have much higher variability, making it more difficult to determine the amount of sensitivity loss. One of the objectives of clinical visual field testing is to utilize methods that minimize the amount of variability in perimetric results.
The physiologic basis for perimetry
One of the advantages of perimetry and visual field testing is that the pattern, shape, and location of sensitivity losses reflect the anatomical arrangement of the visual pathways Fig. 35.2 presents a basic cross-sectional view of the visual pathways from the eye to the visual centers of the brain. It is important to note that the temporal visual field projects to the nasal portion of the retina, the nasal visual field is represented in the temporal retina, and the superior visual field projects to inferior retina and inferior visual field is imaged on the superior retina. All designations in this chapter are with reference to the visual field location rather than the retinal area. The optics of the eye form an image on the retina, where light intensities are converted into electrical impulses by approximately 100 million photoreceptors (rods and cones), which transmit the electrical signals to neural mechanisms in the inner retina (bipolar, horizontal, amacrine, inter-plexiform, and retinal ganglion cells). The optic nerve consists of retinal ganglion cell axons that then leave the eye and travel back to the brain. The ganglion cell fibers from the temporal retina (representing the nasal visual field) are shown in red in Fig. 35.2 and remain on the same side, while the retinal ganglion cell fibers from the nasal retina (representing the temporal visual field) are shown in blue and cross over to the opposite side at the optic chiasm. At this point, the information from both eyes is combined so that the right half of the visual field processing is sent to the left hemisphere and the left half of the visual field information goes to the right hemisphere. The fibers terminate in the lateral geniculate body in the thalamus, and from there fibers from the lateral geniculate are sent to primary visual cortex. Between the optic chiasm and primary visual cortex, the fibers are still arranging themselves to be able to produce a point-by-point mapping of the visual field for both eyes. This anatomical arrangement provides clinically useful information in determining the locus of damage to the visual pathways. A highly detailed description of the anatomy and physiology of the visual system may be found elsewhere in this volume, as well as in Webvision: The Organization of the Retina and Visual System .

Common forms of perimetric testing
Usually, three primary methods of visual field testing are performed clinically: (1) static perimetry, (2) kinetic perimetry, and (3) suprathreshold static perimetry. These procedures have specific advantages and disadvantages, and each can be useful for clinical evaluation. In this view, these procedures should be regarded as complementary and fulfilling particular requirements for diagnostic purposes. Selection of which procedure to use depends on the capabilities, attention and cooperation of the patient, and the relative importance of perimetry as part of the eye examination.
Static perimetry
The most common form of visual field testing conducted by automated perimeters is static perimetry, consisting of detection of a small white stimulus superimposed on a uniform white background at various locations in the field of view. At each location, the minimum amount of light needed to detect the stimulus is determined and is referred to as the differential or increment sensitivity threshold. The pattern of stimulus locations corresponds to either a polar coordinate system (radius and meridian) or a Cartesian coordinate arrangement (a grid bracketing the horizontal and vertical meridians). A number of test procedures have been developed for measuring threshold sensitivities (method of limits, staircase, Swedish Interactive Threshold Algorithm [SITA], ZEST, TOP, GATE). In addition to the sensitivity threshold measurements, procedures for estimating test reliability (false positives, false negatives, fixation losses, alignment [eye and head tracking]) and variability are also included.
Visual field results are typically presented in a composite framework that includes demographic information and testing conditions, a graphical representation of visual field sensitivity (grayscale or color map), general or widespread sensitivity in comparison to an average normal person of the same age, an assessment of localized sensitivity loss, alignment during the test (eye and head tracking), and summary statistics that provide the amount of diffuse loss, localized damage, consistency, reliability, compatibility with glaucomatous deficits, and stage of visual field normality. Each device has its own terminology and analysis methods for providing this information. Fig. 35.3 presents a representative print out of visual field results obtained using a Humphrey field analyzer, and Fig. 35.4 demonstrates a similar arrangement for the Octopus perimeter. An example of grayscale representations in Fig. 35.5 is presented for a normal visual field (A), a visual field with widespread or diffuse sensitivity loss (B), and localized sensitivity reductions (C). Note that the grayscale or color map representation provides a useful method for evaluating the pattern and shape of visual field sensitivity characteristics.



Among the advantages of automated static perimetry are the use of a standardized test procedure, the ability to easily exchange information from one device and office to another, comparisons to age-corrected normal population characteristics for a single visit or for changes over time, methods for monitoring response reliability, eye and head tracking, and assessments of variability within and between tests. Also, database programs such as FORUM for the Humphrey field analyzer allow visual field information to be stored concurrently with retinal photographs, optical coherence tomography results, intraocular pressure, and other relevant information obtained during a clinic visit. Disadvantages of current automated static perimetry tests include limitations in the ability to efficiently evaluate the entire field of view, less flexible test procedures that are not adaptive, a significant burden on the patient’s continual attention and performance throughout the test, higher variability in damaged visual field regions, and limited interactive capabilities between the patient and the examiner.
Kinetic perimetry
Kinetic perimetry consists of moving a stimulus of predetermined size and intensity on a uniform background luminance to determine the locations at which the stimulus can first be detected. Although this form of visual field testing was the preferred method approximately 40 years ago by presenting small stimuli on either a dark 1- or 2-meter tangent screen or a lit bowl perimeter, it is not used as frequently today. Only a limited number of older practitioners are able to perform this test manually, and only partial forms of kinetic perimetry are available on a few automated perimeters. To become proficient in conducting kinetic perimetry requires a considerable amount of time (6–12 months), practice, and experience, with most of the training mentored and hands-on. An excellent description of how to perform kinetic perimetry is found in the book authored by Anderson.
Most kinetic perimetry is conducted using either a black tangent screen (1 or 2 meters) or a Goldmann or Goldmann-like perimeter with the examiner performing the test manually. A few automated devices have a limited kinetic perimetry test, and the Octopus 900 perimeter has the most advanced semiautomatic kinetic perimetry test. The visual field (hill of vision) is mapped by moving a stimulus of specified size and luminance from the periphery toward the central fixation point along radial meridians until the patient responds when they can first detect the stimulus. The rate of motion should be constant and is typically 4 to 5 degrees per second for locations in the far periphery, and 1 to 2 degrees per second for more-central visual field locations. Once these kinetic scans have been made for meridians around the visual field, the detection points are connected by lines that denote an isopter (region of equal sensitivity). In a normal eye, isopters are elliptical, with the long axis in the inferior temporal quadrant and the short axis in the superior nasal quadrant. Departures from the egg-shaped normal isopter require additional scans to properly characterize the contour of the isopter. Multiple isopters can be derived by using different stimulus size and luminance combinations. Areas of nonseeing (scotomas) are mapped by placing a stimulus near the center of the deficit and moving outward from the center in all directions to define the outer contour of the scotoma. To evaluate visual field locations between isopters to search for possibly scotomas, static spot checks are performed by briefly flashing a stimulus at various locations. When the visual field has been completely evaluated, a two-dimensional map of sensitivity to light is produced. A representative example of a kinetic perimetry test result for a normal healthy right eye is presented in Fig. 35.6 .

Kinetic perimetry used to be the predominant method of visual field testing about 40 years ago, but with the advent of automated perimetry, static testing is now the primary method. However, kinetic perimetry is still a valuable technique for assessing visual field status in patients with retinal or neuro-ophthalmologic conditions, and for patients with limited attention spans or cognitive impairment. There are distinct advantages and disadvantages associated with kinetic perimetry. The test is highly interactive between the examiner and the patient, so flexible test procedures can readily be implemented and adjusted throughout the test procedure. Kinetic perimetry testing permits accurate mapping of the location, pattern, and shape of visual field losses to assist in differential diagnostic evaluation. The far peripheral visual field can be assessed in a much more efficient manner when compared with static perimetry. The disadvantages of kinetic perimetry include a greater amount of variability when compared with static perimetry, large individual differences among examiners in their technique, lack of standardized procedures, limited normal population characteristics, and difficulty in properly representing widespread or diffuse visual field sensitivity loss. Whereas static perimetry has a well-defined procedure and may be likened to the types of strategies used in the game of checkers, kinetic perimetry is a heuristic, interactive series of strategies that may be more representative of chess. It is probably for this reason that there has been limited implementation of kinetic test strategies for automated perimetry.
Suprathreshold static perimetry
Although there have been significant advances in deriving more efficient test strategies for static and kinetic perimetry, they still require more time to achieve quantitative perimetry results compared with a rapid screening to determine whether the visual field is within or outside normal limits. For a general eye examination or for population-based screening, it is desirable to have a very efficient test procedure that also has good performance. The basic strategy behind suprathreshold static perimetry is to present stimuli at key locations in the visual field using stimuli that should be easily seen by individuals with normal visual function (normal versus abnormal visual field) or to further characterize areas of loss as being mild, moderate, or advanced. Unfortunately, there have been some drawbacks to determining optimal suprathreshold static test procedures: (1) the vast majority of test procedures that have undergone rigorous evaluations have been quantitative static and kinetic visual field test procedures rather than visual field screening tests, with a few exceptions ; (2) the information concerning performance characteristics of suprathreshold static perimetry is quite limited; (3) whereas there are many potential suprathreshold static test procedures, it is not known which provides better performance, and there are few comparisons of various methods; (4) quantitative static perimetry test procedures have become more efficient, reducing the advantage of condensed testing time with suprathreshold static perimetry; and (5) properties of the test procedures (underlying assumptions, number of locations tested, stimulus properties, test strategy, guidelines for interpretation of results, etc.) vary widely from one test to another. Therefore, it is of interest to note that frequency doubling technology (FDT) has two screening tests (one with higher specificity and one with higher sensitivity) that have good performance and can provide a screening of a normal eye in less than 30 seconds and an eye with visual field loss in 1 to 1.5 minutes.
Suprathreshold static perimetry provides a standardized test procedure that is efficient and is quite suitable for screening purposes, either within a clinical office environment or for large-scale population-based screening. It can also be used to provide an assessment of the entire visual field. However, it provides only limited quantitative information. An example of a suprathreshold static perimetry result for the right eye obtained using the two-zone 120 location test on the Humphrey field analyzer is presented in Fig. 35.7 .

Detection of sensitivity loss and interpretation of results
Information for evaluating and interpreting the results of a single visual field test can be found on the printed output of the test results. Fig. 35.8 presents the results of a visual field test performed on the Humphrey field analyzer using the 30-2 SITA Standard test procedure to assess the left eye of a patient with superior and inferior partial arcuate glaucomatous visual field defects.

On the top portion of the visual field printout is the patient’s name, eye that was tested, the patient ID code, and date of birth. Below this are listed the test conditions that were used (background luminance, stimulus size, fixation target, fixation monitoring method, etc.), the test strategy, reliability characteristics (false-positive responses, false-negative responses, fixation losses), and the patient’s age and ocular conditions (distance refraction, pupil size and best-corrected visual acuity). This listing makes it possible to immediately determine whether proper testing conditions were employed, whether the test was reliable, and whether other information (distance refraction, visual acuity, date of birth, etc.) is correct.
If there are excessive false-positive responses (pressing the response button when no stimulus was presented), this indicates that the patient was pressing the button too frequently (“trigger happy”), and it is likely that there will also be excessive fixation losses and extremely high (nonphysiologic) threshold sensitivity values. If the patient becomes drowsy or inattentive, a high number of false negatives (not pressing the response button when a stimulus 9 to 10 times greater than a previously determined threshold value) can occur. However, it should also be noted that if the patient has more advanced visual field loss, the damaged areas will have higher variability, which can also result in high false negatives.
Below this information is the visual field data. The foveal threshold sensitivity value is presented, along with a probability level if it is below the lower 5%, 2%, 1%, or 0.5% of values for a normal individual of the same age. This is followed by an X/Y plot of threshold sensitivity values in decibels (dB) at each of the visual field locations on the left, and a grayscale representation of visual field sensitivity on the right. The decibel scale is a relative logarithmic scale (log threshold sensitivity ×10). A value of 0 dB is defined as the maximum luminance intensity that the Humphrey field analyzer can produce (10,000 apostilbs, or 3175 cd/m 2 ). The gray scale provides an interpolated two-dimensional graphic of the pattern and shape of visual field deficits for differential diagnostic purposes. (It should be noted that this scale is coarse, and subtle defect patterns are often not visualized, for example, subtle defects that are shown on the total and pattern deviation plots, discussed further on.) The lower left graphs present the numerical deviation of threshold sensitivity values from the average normal observer of the same age at each visual field location (top graph) and the probability that it is lower than the 5%, 2%, 1%, or 0.5% probability of the normal population (bottom graph). On the top graph, positive numbers reflect threshold sensitivity values that are above the average normal of the same age, and negative numbers indicate threshold sensitivity values that are below the average normal. The lower graph with probability levels demonstrates the locations with reduced sensitivity and the magnitude of this deficit. This data representation is referred to as the Total Deviation plot.
Visual field sensitivity loss can be widespread (diffuse or generalized) or it can be localized to a specific region. To partially disconnect the widespread and localized visual field loss components, the Humphrey field analyzer adjusts the individual threshold sensitivity values by the general height value. The general height is determined by ranking all the threshold sensitivity values from highest to lowest and selecting the value corresponding to the 85th percentile (for the 24-2 pattern, this is the seventh-highest threshold sensitivity value). All the threshold sensitivity values are adjusted by this value, which diminishes all or most of the widespread sensitivity loss to reveal the localized deficits. The deviation values and probability levels are similar to those of the Total Deviation plots, and this representation is referred to as pattern deviation. Fig. 35.9 presents examples of the clinical benefit of comparing the total deviation (left) and pattern deviation (right) plots to illustrate: (A) a right eye of a trigger-happy patient where total deviation values are normal and pattern deviation is outside of normal, (B) a right eye of a patient with widespread or diffuse loss where total deviation is outside of normal and pattern deviation is mostly within normal limits, (C) a superior arcuate deficit for a right eye that depicts localized loss, and (D) a left eye that has a combination of both localized and widespread or diffuse loss.

This point-by-point characterization contains a substantial amount of information, so general summary statistics are also available. Mean deviation (MD) is the average deviation of threshold sensitivity values from the average normal individual of the same age. However, not all locations have the same effect, as more-central visual field locations are given more weight than ones that are peripheral. Pattern standard deviation is the amount of irregularity or perturbation of the normal slope of the visual field that is calculated as the root mean square deviation. Areas of visual field sensitivity loss are the primary sources responsible for producing deviations from the normal slope. Most visual fields are performed on glaucoma patients, so there is also an analysis that is designed for identifying visual field sensitivity loss patterns that are characteristic of glaucoma. The glaucoma hemifield test (GHT) contains five superior and five mirror image inferior clusters of visual field locations that mimic the pattern of losses that typically occur with glaucoma. It then compares the asymmetry between the average sensitivity for each cluster for mirror image regions in the superior and inferior visual field. There are only minor differences in the average values for superior and inferior clusters for normal healthy visual fields. However, visual field losses produced by glaucoma will result in values that are often outside of the normal asymmetry values. There are five designations that are presented as a result of this analysis: (1) within normal limits, if all cluster asymmetries correspond to normal values; (2) outside normal limits, if one or more of the cluster comparisons is outside of the 1% probability level for normal; (3) borderline, if one or more of the cluster comparisons are outside of the 3% probability level; (4) depressed sensitivity, if the overall threshold sensitivity values are below the lower 0.5% probability level; and (5) abnormally high sensitivity, if the overall threshold sensitivity values are above the upper 0.5% probability level. Fig. 35.10 presents the five superior and five inferior clusters represented in a 30-2 test presentation pattern.

The visual field index (VFI) is the final summary statistic, which determines the percentage of the visual field that is within normal limits by comparing the visual field to a population of normal healthy eyes. A normal visual field has a VFI value of 100% and a perimetrically blind visual field has a VFI score of 0%. This can then provide a simple value that can be used to track the rate of change over time. To minimize the influence of cataract on the VFI that would result in widespread or diffuse loss, only points that are represented as beyond normal limits for the Pattern Deviation plot are considered. Additionally, greater weight is provided to more-central points than peripheral points in accordance with cortical magnification factors. Although this is a simple representation of overall visual field status, it should be kept in mind that it has both ceiling and floor effects. – Not only do normal visual fields have a VFI of 100%, but small deficits where the MD is better than –5 or –6 dB will also elicit a VFI of 100%, which results in a ceiling effect. When there is advanced glaucomatous visual field loss, the raw dB sensitivity values are beyond the range of pattern deviation values, so the algorithm switches to total deviation probabilities. This produces a discontinuity in the VFI values that represents a floor effect and makes it difficult to determine a rate of progression.
Finally, at the bottom of the visual field printout is a graph of gaze tracking that monitors the patient’s alignment with the perimeter and fixation accuracy. Gaze tracking is a most valuable component because it provides the examiner with valuable information about the patient’s performance during the test. Gaze tracking uses infrared light that will not interfere with the test procedure and uses a video camera to capture the location of the first Purkinje image (corneal reflex) in relation to the edges of the pupil (retinal red reflex). The gaze tracking graph presents the status of alignment throughout the test procedure. By examining this graph, it is possible not only to determine the quality of alignment and fixation throughout the procedure but also to provide an indication of droopy upper eyelids, inattention and fatigue, excessive blinking and dry eye, and consistency of alignment. Upper bar deflections indicate departures from fixation, using three bar sizes of small (approximately 2–4 degrees of fixation loss), medium (about 4–6 degrees of fixation loss), and large (>6 degrees of fixation loss). Lower bar deflections indicate interruptions of the infrared beam reflections (e.g., blinks) or dry eye (tear film breakup). Fig. 35.11 presents several examples of gaze tracking: (A) good gaze tracking and accurate fixation; (B) excessive blinking or dry eye; (C) droopy eyelid or fatigue; (D) inattention and fatigue near the end of the test; and (E) generally poor alignment throughout the test.

Overall, the single visual field analysis that is produced on the printed output contains a wealth of information concerning visual field status, interpretation of results and comparison with a healthy normal population, reliability, alignment, and other properties that can be most helpful for clinical assessment. It is important to keep in mind that everything that is provided on the visual field printout is important—it is not useful to only concentrate on selected portions of the output. It is also helpful to remember that visual field testing is only one of many items that are part of the eye examination.
Visual field loss patterns created by various pathologies
Careful clinical assessment of the pattern and shape of visual field deficits is probably the most critical component of visual field interpretation. Although printed output of the visual field is two-dimensional, it is important to keep in mind the three-dimensional properties of the visual field (length, width, and depth). There are many features that can provide clinical insights into the disease process and locus of involvement of the visual pathways, including the pattern and shape of areas of sensitivity loss, whether the transition from normal to impaired areas has steep or gradual boundaries, and whether the deficit “respects” (terminates abruptly) at specific locations that correspond to anatomical properties of the visual pathways. Fig. 35.12 presents a general representation of damage to fibers at different location in the visual pathways (blue lines) and the resulting patterns of visual field loss that are associated with this impairment. More detailed examples of visual field losses resulting from injuries to specific portions of the visual system may be found in other literature sources.

It is critical to have a systematic approach to use for interpreting the visual field. In this view, the next segment of this chapter provides a listing of key pattern and shape characteristics of visual field losses, followed by a set of sequential guidelines for performing the assessment of a single visual field.
Patterns of visual field loss
- •
Generalized or widespread visual field sensitivity loss . This is a nonspecific type of visual field loss because it can be produced by a variety of sources (ocular media, small pupil, visual pathology to many regions of the visual pathways, cognitive and attentional lapses).
- •
Visual field constriction . This is a narrowing of the field of vision that is often referred to as tunnel vision, due to its characteristic shape. A variety of insults to the visual pathways can create a constricted visual field.
- •
Ring scotomas . These losses tend to occur in the midperipheral visual field as a total or partial ring of sensitivity loss surrounded by regions of higher sensitivity. They are typically produced by retinitis pigmentosa or another type of retinal degeneration.
- •
Central scotomas . In general, these deficits are produced by damage to mechanisms in the macular region or by certain types of optic neuropathies.
- •
Arcuate scotomas . These defects are produced by damage to the arcuate nerve fiber bundles that are produced by glaucoma, other optic neuropathies, or vascular insults (branch artery occlusion), as the vascular supply of the eye travels in an arcuate fashion.
- •
Nasal steps . This can be regarded as a part of the arcuate defect that has a distinct sharp transition from seeing to nonseeing at the horizontal meridian. It most commonly occurs in glaucoma.
- •
Defects that “respect” the horizontal meridian . This is a sharp boundary between seeing and nonseeing at the horizontal meridian on the nasal side of the visual field, and is usually associated with glaucoma, other optic neuropathies, or branch artery occlusions.
- •
Centrocecal scotomas . This pattern denotes visual damage that includes the blind spot (ceco) and fixation. This deficit has a candle flame shape and is associated with damage to fibers in the papillomacular bundle that subserve the macula and the region between fixation and the optic disc. Most optic nerve fibers are contained in the papillomacular bundle. Some optic neuropathies and macular damage can produce this deficit.
- •
Defects that “respect” the vertical meridian . The fibers conveying information from the left and right sides of vision for both eyes separate at the central vertical meridian or midline when the information from both eyes is combined at the chiasm and after the chiasm. Damage to nerve fibers at the chiasm and beyond results in a steep, abrupt transition in sensitivity at the vertical meridian.
- •
Bitemporal visual field deficits . A bitemporal visual field deficit that respects the vertical meridian is characteristic of damage to the optic chiasm.
- •
Visual field losses that resemble a horizontal wedge . This is a very uncommon visual field deficit in which the area of visual field loss resembles a horizontal wedge or tongue along the horizontal meridian or a tongue of remaining visual field sensitivity along the horizontal meridian. When homonymous, this pattern corresponds to damage of neural fibers at the level of the lateral geniculate body. Bitemporal horizontal wedge defects along the horizontal are usually congenital in origin.
- •
Homonymous visual field loss . Visual field losses that occur on the same side of vision (left or right) for both eyes are homonymous (the nasal visual field of one eye combined with the temporal visual field of the other eye). The vertical meridian is respected, and the visual pathway damage is in the optic radiations posterior to the optic chiasm. A total homonymous hemianopsia (complete homonymous loss of vision for the left or right visual field) indicates that all of the fibers in the optic radiations have been damaged at some location after the optic chiasm.
- •
Congruous and incongruous visual field loss . As a rule, the greater the amount of incongruity of loss between the two eyes (i.e., one eye has more a more extensive deficit than the other eye), the closer the damage is to the optic chiasm. Deficits to the optic radiations in the optic tract produce incongruous visual field deficits between the two eyes, whereas damage to the optic radiations in the temporal and parietal lobe are semicongruous. Primary visual cortex lesions produce visual field deficits that are highly congruous.
- •
Pie in the sky visual field deficits . A homonymous semicongruous visual field deficit the appears as if a piece of a pie is missing from the superior visual field that respects the vertical meridian is typically associated with temporal lobe lesions, in accordance with the arrangement of the optic radiations traveling through the temporal lobe.
- •
Pie on the floor visual field loss . Conversely, a semicongruous homonymous visual field deficit that respects the vertical meridian that resembles a missing piece of pie that has fallen to the floor is usually associated with parietal lobe damage to the optic radiations traveling through the parietal lobe.
- •
Cookie cutter punched-out deficits . Visual field losses that appear to be nearly identical between the two eyes, as if a hole punch or cookie cutter had removed a portion of the visual field is indicative of an occipital lobe (primary visual cortex) deficit.
Although there will sometimes be exceptions to these general principles, these guidelines will be helpful in properly interpreting visual field information and determining the most likely locus of damage to the visual pathways. There are many excellent sources that provide examples of visual field deficits associated with damage to specific regions of the visual pathways.
Visual field interpretation guidelines
- 1.
Place the visual field printout of the left eye on the left and the right eye on the right.
- 2.
Determine whether the appropriate test was performed, the date of birth is correct, pupil size is 3 mm or greater, visual acuity is listed, the near refractive correction is appropriate, the tests were reliable, and the results are consistent with the status of the visual pathways are not artifactual (droopy lid, small pupil, lens rim, etc.).
- 3.
For each eye, evaluate whether the visual field is within normal limits or has sensitivity loss.
- a.
If the visual field is reliable and within normal limits for both eyes, the assessment is complete.
- b.
If one or both visual fields are unreliable or there are eye and head tracking problems, determine whether the tests should be repeated.
- a.
- 4.
If there is evidence of sensitivity loss, determine whether it is just one eye or both eyes that have evidence of impairment.
- a.
If the loss is in just one eye, then the damage occurred prior to the location where the information from both eyes is combined (optic chiasm), indicating that the deficit is produced by damage to the retina or the optic nerve, or by ocular media problems.
- b.
If the visual field loss is in both eyes, then the damage may be present at the chiasm or postchiasmal, but it can also be due to retinal or optic nerve damage in both eyes.
- a.
- 5.
What is the general location of the loss (superior, inferior, nasal, temporal)? If the loss is extensive, where is the most prominent region of visual field loss?
- 6.
What is the shape and location of the visual field deficit (central, centrocecal, arcuate, nasal step, bitemporal, quadrant, hemifield, constricted, respect the horizontal meridian, respect the vertical meridian)?
- 7.
How do deficits from the two eyes compare to each other?
- a.
Is the deficit binasal (probably glaucoma, optic neuropathy, or retinal disease)?
- b.
Is the deficit bitemporal (chiasmal lesion)?
- c.
Is the deficit homonymous (same side of vision)?
- i.
Is it a total homonymous hemianopia (all visual pathway fibers are damaged past the chiasm)?
- ii.
If it is not a total homonymous hemianopia, is it a quadrant deficit?
- iii.
How congruous is the deficit between the two eyes? (The more congruous, the farther back the damage is present in the visual pathways.)
- iv.
What is the shape of the area of loss? Pie in the sky (deficit in the superior visual field) is most common for temporal lobe deficits, while pie on the floor (deficit in the inferior visual field) occurs more often with parietal lobe lesions.
- i.
- a.
- 8.
Once this has been completed, the visual field interpretation should be combined with other clinical findings to determine a final lesion localization and differential diagnosis.
Box 35.1 provides a brief summary of the visual field interpretation guidelines.
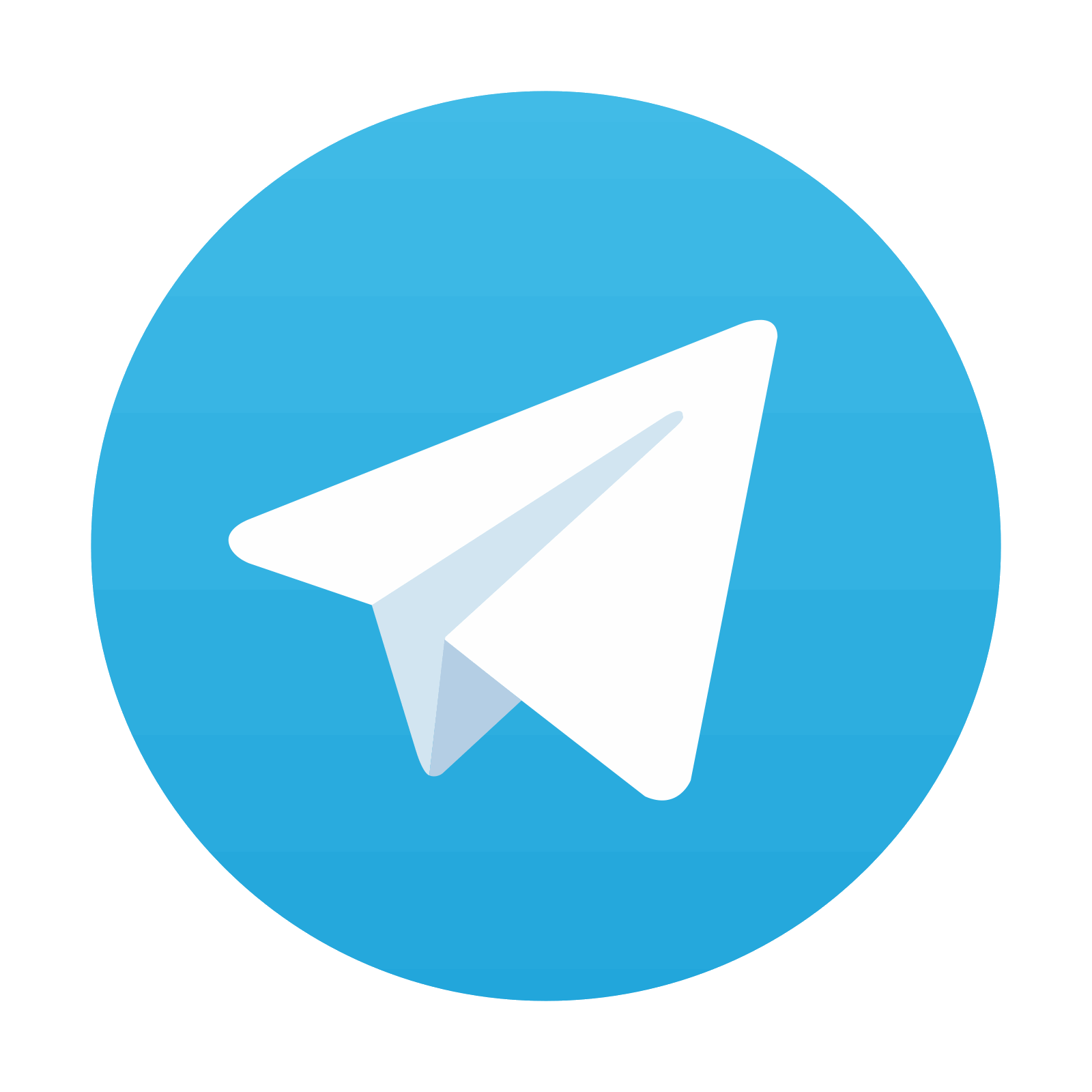
Stay updated, free articles. Join our Telegram channel
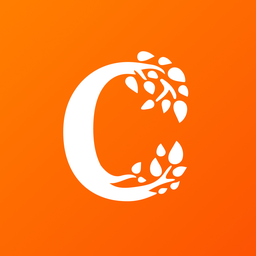
Full access? Get Clinical Tree
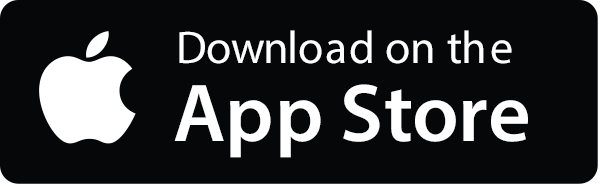
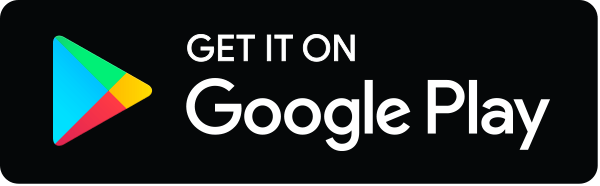
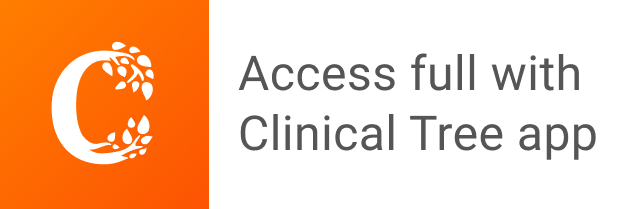