The basic architecture, signal flow, and neurochemistry of signaling through the vertebrate retina is well understood: photoreceptors, bipolar cells (BCs), and retinal ganglion cells (RGCs) are all thought to be glutamatergic neurons and the fundamental synaptic chain that serves vision is photoreceptor → BC → RGC. Detailed signaling network topologies and synaptic mechanisms are far from complete for any retinal network, but new technologies are rapidly fleshing out key network motifs and molecular mechanisms. For example, RGCs express different mixtures of ionotropic glutamate receptors (iGluRs) and each receptor can be composed of many different subunits, leading to a vast array of possible functional varieties. At a larger scale, network topologies are too numerous to resolve with current physiologic or pharmacologic data. Each RGC contacts many different amacrine cells (ACs) and a full description of the inputs to any given RGC does not yet exist. Physiology can screen only a limited parameter space for any cell. Even so, new systems of connectivity have emerged from connectomics data that can guide physiologic analyses. Pharmacology remains a fluid field with many incomplete tools, and an immense diversity of neurotransmitter receptor subunit combinations, modulators, and downstream effectors remains to be screened for any cell type. Morphology, augmented by immunochemistry and physiology, remains the core tool in discovering new details of retinal organization. Nothing has been as powerful as transmission electron microscopy for discovering retinal networks. Mammalian night (scotopic) vision is a prime example. Its unique pathways were described by Helga Kolb and E.V. Famiglietti Jr. using electron microscopy. Subsequent physiological analyses provided clarification of how the network functions but would not have yielded the correct network architecture. Further complexities of the rod pathway have been discovered by anatomical studies, including the fact that the network rewires in retinal degenerations ( Box 21.1 ). In the past decade, new high-throughput electron imaging has provided new information about retinal circuitry. , The extensive advances in the molecular bases of retinal development and new findings in neuroplasticity are beyond the scope of this chapter. However, many powerful new tools have revealed connections we have long considered as static or hard-wired in the retina display many of the same molecular attributes as plastic pathways in brain.
- •
The retina expresses mGluR6 receptors in both rod and cone ON bipolar cells (BCs).
- •
The human mGluR6 gene GRM6 is expressed predominantly in retina.
- •
The mGluR6 receptor is sign-inverting.
- •
The mGluR6 receptor dominates scotopic vision but may not be essential for photopic vision.
- •
Some human night-blind patients have GRM6 mutations with no photopic deficit.
- •
The mGluR6 drives blue ON center cone BCs and retinal ganglion cells (RGCs).
- •
fMRI in rodents shows that blockade of mGluR6 receptors attenuates blue-driven signals in the Lateral Geniculate Nucleus.
- •
Simultaneously, blue-driven cortical signals are enhanced, suggesting that blue percepts are effectively carried by OFF channels.
- •
OFF center BCs can support photopic vision.
The basic signal flow in retina is overlaid on a well-studied cell architecture ( Fig. 21.1 ). Retinal ON and OFF BC polarities are generated in the outer plexiform layer and mapped onto the inner plexiform layer (IPL) into largely separated zones. The distal sublamina a receives inputs from OFF BCs and therein the dendrites of OFF RGCs collect signals via BC synapses. The proximal sublamina b receives inputs from ON BCs and therein the dendrites of OFF RGCs collect signals via BC synapses. ON-OFF RGCs thus collect inputs from both sublayers. A key variation on this simple notion of the IPL comes from nonmammalian retinas in which many cone BCs show synaptic swellings throughout the IPL, implying that ON BCs could multiplex some of their signals into sublamina a. Combinations of electrophysiology, dye injection, and computational transmission electron microscopy have now shown that mammalian cone ON BCs provide a nominal en passant input to unusual cells (e.g., dopaminergic axonal cells [AxCs]) that arborize in sublamina a, yet show distinct ON responses. This sophistication indicates that the primary sublamination of the IPL, although profound and important for segmenting signal flow to the brain, does not prevent the emergence of exceptions, as we will note further.

Kinds of neurons
The retina is a thin, multilayered tissue sheet—an image screen—containing three developmentally distinct, interconnected cell groups that form signal-processing networks:
Class 1, sensory neuroepithelium (SNE): photoreceptors and BCs
Class 2, multipolar neurons: RGCs, ACs, and AxCs
Class 3, gliaform neurons; horizontal cells (HCs)
These three cell groups comprise over 60 to 70 distinct classes of cells in mammals (Jones et al., 2008) and well over 100 to 120 in most nonmammalian retinas, all assembled into multiple pathways into the brain.
The Sensory Neuroepithelium (SNE) phenotype
The SNE phenotype includes photoreceptors and BCs. These cells are polarized neuroepithelia with apical ciliary-dendritic and basal axonal-exocytotic poles. They form the first stage of synaptic gain in the glutamatergic photoreceptor → BC → RGC → CNS vertical chain. This aggregates photoreceptor signals into BC receptive fields and amplifies their signals. The basal ends of the BCs form the IPL. There are at least 12 kinds of BCs in mammals , and BCs delimit different functional zones in the IPL, suggesting nearly 1 micron precision in lamination. Both photoreceptors and BCs use high fusion–rate synaptic ribbons as their output elements, fueled by hundreds to thousands of nearby vesicles. The retina is the only known tissue in which SNE cells are arrayed in a serial chain.
As summarized in Fig. 21.2 , most mammals possess three classes of photoreceptors: rods expressing rhodopsin 1 (RH1) visual pigments, blue cones expressing short wave–system 1 (SWS1) visual pigments, and green cones expressing long wave–system green (LWSG) visual pigments. Conversely, the most visually advanced and diverse vertebrate classes (teleost fish, avians, reptiles) possess up to seven known classes of photoreceptors (RH1 rods; SWS1 UV/violet cones; SWS2 blue cones; LWSR and RH2 green members of double cones; and LWSR and RH2 green single cones).

Similarly, the diversity of BCs in mammalians is lower (10–13) than nonmammalians (>20). This reduced diversity is a result of the Jurassic collapse of the mammalian visual system, when over half of the visual pigment genes, half of the neuronal classes, and almost two-thirds of the photoreceptor classes were abandoned to exploit nocturnal niches. In addition, the disproportionate proliferation of rods in the mammalian retina was accompanied by the loss of mixed rod-cone BCs in mammals and their replacement with pure rod BCs. How this occurred is unknown, but it could not have been due to an absolute selectivity of rod BCs for rods, as they will readily make contacts with cones when rods are lost in retinal degenerations. As we will see, the mammalian retina has exploited a re-entrant use of synapses to enhance scotopic vision. The relationship between BCs and photoreceptors is still unclear, but there is both anatomical and molecular evidence that BCs were initially photoreceptors. For example, many nonmammalians possess BC Landolt clubs, which are apical extensions extending from a BC primary cilium, extending past the outer plexiform layer into the outer nuclear layer and containing packets of outer segment–like membranes. Whether they are photosensitive has never been determined. Further, SWS1 blue cones and cone BCs share some SWS1 cis -regulatory sequences.
The multipolar neuron phenotype
The multipolar neuron phenotype (Jones et al., 2008) includes ACs, AxCs, and RGCs. Multipolar neurons can be further divided into axon-bearing (RGCs, AxCs) and axonless cells (ACs). Mammals display around 30 kinds of ACs. The 15 to 20 kinds of mammalian RGCs , are classical projection neurons. RGCs are postsynaptic at their dendrites and presynaptic at their axon terminals in CNS projections. So far, all are presumed to be glutamatergic. ACs are local circuit neurons similar to periglomerular cells in the olfactory bulb. ACs lack classical axons and often have both presynaptic and postsynaptic contacts on their dendrites, although some ACs partition inputs and outputs into different parts of their dendritic arbors. Most ACs are GABAergic and the remainder are glycinergic. Several classes of ACs are dual transmitter cells, expressing both acetylcholine and GABA; serotonin and GABA (in nonmammalians); or peptides and GABA or glycine. In between are the AxCs, also known as polyaxonal cells and intraretinal RGCs, which have distinct axons that project within the retina. One dramatic example of the AxC phenotype is the TH1 dopaminergic AxC. This cell releases dopamine at unknown but probably axonal sites and likely glutamate at others (Jones et al., 2008), similar to nigrostriatal neurons. Some polyaxonal cells are GABAergic. There is no evidence for a glycinergic AxC. Multipolar neurons are characterized by numerous neurites branching in the plane of the retina, most collecting signals from BCs. Multipolar neurons are among the earliest to develop in the retina and quickly define the borders of the IPL and its stratifications. Multipolar neurons all manifest somewhat classical “gray”-like synapses, generally with small clusters of less than 200 vesicles.
The gliaform cell phenotype
This phenotype contains the HCs, the somas and processes of which are restricted to the outer plexiform layer. Although HCs are multipolar and neuron-like, and may display axons, they do not spike. Further, they express many glial features such as intermediate filament expression and very slow voltage responses. Further, HCs produce high levels of glutathione and make direct contact with capillary endothelial cells in some species, suggesting they play homeostatic roles similar to glia. Even so, HCs clearly mediate a powerful network function, collecting large patches of photoreceptor input via AMPA receptors and providing a wide-field, slow signal antagonistic to the vertical channel. The mechanism of HC antagonism remains a matter of uncertainty and debate. HCs do make conventional-appearing synapses onto neuronal processes in the outer plexiform layer, and in fishes these synapses are made onto dendrites of glycinergic interplexiform cells, a form of AxC. However, these are so sparse in all species and contain so few vesicles that they cannot be the source of the large, sustained opponent surrounds of retinal neurons that HCs generate. HCs must use some other mechanism. The phylogenetics of HCs has been thoroughly reviewed. HCs in mammals are postsynaptic to cones at their somatic dendrites. One class of HCs common in mammals (foveal type I in primates, type A in rabbits and cats, and absent in rodents) contacts cones alone. A second class of HCs (extrafoveal type I in primates, type B in rabbit and cats, and the only known HC in rodents) displays axons several hundred microns long that branch profusely and form massive arborizations contacting hundreds to thousands of rods. Another class of primate HC (type II) has axon terminals contacting cones and rods. Importantly, the axon of HCs appears to be electrically inactive, and these somatic and terminal regions are believed to act independently. HCs also appear to be early-developing pioneer cells that define the outer plexiform layer. After the RGCs and HCs define the layout of the inner and outer plexiform layers respectively, photoreceptors and BCs mature and search for connections.
Novel cells
Ramon y Cajal’s extensive analysis of retinal neuronal includes a number of unusual cell morphs yet to be analyzed by modern techniques. Indeed, it is likely that many of the cells he saw were never published. Recently, two candidate novel cell classes have been described. Della Santina et al. (2016) used visualization of the fluorescent protein cerulean driven by the OFF BC promoter VsX1 to discover a set of monopolar interneurons that resemble the proximal axonal part of a BC but lack dendrites. These cells are termed glutamatergic monopolar interneurons (GluMIs) and are obviously part of the BC lineage. They target RGCs and are driven by ACs. Another BC-like neuron has been described by Young et al. and termed a Campana cell . Its soma is embedded in the AC layer, but it is clearly bipolar in morphology. Pharmacologic analysis of its light responses suggest that it is driven by both rods and cones, generating largely typical ON responses via mGluR6-like mechanisms, but there may be ON BC inputs on its axonal field as well. The cells are very sparse but appear to drive RGCs.
True glia and vasculature
The neurons of the retina are embedded in an array of vertical Müller glia that span the entire neural retina, forming one-third to one-half of the retinal mass and generating high-resistance junctional seals at the distal and proximal limits of the retina. Most mammalian retinas are vascularized in three capillary beds: at the RGC-IPL border, the AC-IPL border, and the outer plexiform layer. Squirrels (Sciurids) display two beds (at the RGC-IPL and AC-IPL layer borders) and rabbits (Lagomorphs) have none at all, similar to all other nonmammalian vertebrates. The RGC layer of many vascularized species also displays classical astrocytes, although their roles remain unclear. In brain, astrocytes carry out some of the operations attributed to retinal Müller glia, including transport of spillover K + and glutamate, and glucose supply via vascular > glial cell > neuron transcellular transport. Why and how most vertebrate retinas function without vasculature remains uncertain, but it is likely that Müller glia act as a surrogate vascular system with the added ability to accumulate large glycogen stores (like hepatocytes) as part of a glucose-skeleton homeostasis. The segregation of retinal astrocytes away from the IPL remains a mystery.
Basic synaptic communication
With the discovery of the signaling mechanisms of the neuromuscular junction decades ago, one might have thought that the archetypal synaptic format had been discovered. Yet it has become clear, especially in the retina, that every kind of synapse is subtly different, with diverse physics, topologies, and molecular mechanisms leading to very different forms of synapses, most of which do not follow the single presynaptic “bouton” → single postsynaptic target pattern of brain. Further, the arrangement of these systems into synaptic chains in retina is unlike any other known network, including olfactory bulb. In retina, the first stage of synaptic signaling is a direct SNE → SNE synapse ( Fig. 21.1 ): photoreceptor → BC. No other instance of this topology has been discovered in any organism. There are at least six modes of presynaptic-postsynaptic pairing in retina.
Photoreceptor ribbon synapses: small-volume multitarget signaling
It is thought that all photoreceptor signaling is glutamatergic, but sporadic indications of cholinergic physiology and molecular markers have been found in many nonmammalians. Glutamate release from photoreceptors is effected by high rates of vesicle fusion at active sites on either side of a large synaptic ribbon positioned close to the presynaptic membrane. The presynaptic zone is a protrusion or ridge with vesicle fusion sites positioned on the slopes of the ridge (see Fig. 21.4 ). The releasable vesicle pool is so large that photoreceptors and BCs are capable of maintaining continuous glutamate release in response to steady depolarizations. This, among other things, distinguishes photoreceptors and BCs from ACs, which have very small presynaptic vesicle clusters. Although the mechanism is unclear, photoreceptor ribbons accelerate vesicle release and replenishment significantly but are not absolutely required for signaling.
Various vertebrate rods and cones differ greatly in the number of ribbons and postsynaptic targets arrayed within the presynaptic terminals. For example, most mammalian and teleost fish rods have small grape-like presynaptic spherules approximately 3 μm in diameter with a small entrance aperture leading to an enclosed extracellular invagination or vestibule in which thin postsynaptic dendrites are contained ( Fig. 21.3 ). Importantly, glial processes are excluded from the interior of the spherule and any glutamate release must diffuse out of the spherule to reach the Müller glia. However, mammalian rods express the EAAT5 glutamate transporter and likely regulate their own intrasynaptic glutamate levels. Each spherule contains one or two synaptic ribbons and a few postsynaptic targets. In fishes, the postsynaptic targets are the dendrites of roughly five kinds of mixed rod-cone BCs and one kind of rod HC. Thus each ribbon serves no less than six different types of postsynaptic targets. In mammals, only two targets are common: the dendrites of one kind of rod BC and the axon terminals of HCs. There are some instances of sparse OFF BC contact in mammals, but this seems to vary with species and may be an evolutionary relict with variable expression rather than a major signaling pathway. In sum, rod spherules form a sparse-ribbon → small-volume, sparse-target architecture.

Cones and rod terminals in some nonmammalians (e.g., urodele amphibians) adopt a different topology, with the presynaptic ending expanding to form a foot-piece or pedicle some 3 to 5 μm wide. This may be shaped either like a cupola (fishes), the broadly concave interior of which admits some 50 to 100 or more fine dendrites served by roughly 12 synaptic ribbon sites, or like a true pediment (e.g., primate cones), the shallow concavity of which is studded with up to 50 ribbon sites ( Fig. 21.3 ). Cone pedicles in primates target at least 10 different kinds of BCs and at least two kinds of HCs. Mouse cone pedicles are smaller but still target 11 kinds of BCs and one kind of HC. In sum, cone pedicles form a multiribbon → small-volume, multitarget architecture ( Fig. 21.4 ).

BC ribbon synapses: semiprecise target signaling
As with photoreceptors, BC signaling is generally considered glutamatergic, but sporadic exceptions exist. In mammals (especially primates) and amphibians, some BCs contain biomarkers of GABA-related metabolism. In contrast to photoreceptors, BC synaptic endings are topological spheroids, usually multiple (depending on BC type), with dozens to hundreds of ribbons abutting the surface. BCs form no invaginations, so there is no restricted volume into which glutamate is injected by vesicle fusion. In most cases each ribbon is directly apposed to a pair of postsynaptic targets, usually ACs. This is termed a dyad , and although monads, triads, and tetrads do occur, dyads dominate. Large BC terminals such as those found in teleost fishes can drive up to 200 distinct processes. Mammalian BCs drive many fewer targets and most BCs have elaborate, branched terminals with connecting neurites often as small as 100 nm. In contrast to photoreceptors, the targets of BCs are focal. BC terminals are largely fully encapsulated by neuronal processes at their release sites to which they are presynaptic or postsynaptic, with rarely direct contact between the terminal and Müller glia near the synaptic release zone. This means that any glutamate that escapes from the synaptic cleft may travel some distance before glial glutamate transporters can clear it. Thus the potential for glutamate overflow at BC synapses is substantial. This may be particularly important for the activation of NMDA receptors, as they are suspected to be displaced from primary AMPA receptors. Thus, BCs form multiribbon → semiprecise target architectures ( Fig. 21.4 ).
AC and AxC conventional fast synapses: precise presynaptic → postsynaptic signaling
ACs and AxCs are the only retinal cells that make synaptic contacts resembling CNS “gray”-like, nonribbon conventional synapses. ACs target BCs, RGCs, or other ACs. The targets of most AxCs are not well known but appear likely to be ACs and RGCs. Although each AC may make many hundreds of synapses, each synapse contacts only one postsynaptic target, similar to classical multipolar neurons in CNS and spinal cord. The dominant fast transmitters of AC systems are GABA and glycine, with GABAergic neurons making up half to two-thirds of the AC population, depending on species. Additional transmitters such as acetylcholine, peptides, or serotonin (in nonmammalians) are also associated with GABAergic (in most cases) or glycinergic systems. , Acetylcholine (ACh) is a fast excitatory transmitter that is found in paramorphic starburst ACs in mammals and also uses conventional synapses. However, we know of no distinguishing anatomical differences between GABA- and ACh-utilizing synapses in retina.
AC, AxC, and efferent slow transmitter synapses: large-volume signaling
Dopamine (and possibly norepinephrine/epinephrin), as well as peptides in retina, appear to be released by a nonfocal, Ca 2+ -dependent vesicular system without any clear postsynaptic associations. Dopamine and the other slow transmitters likely act via volume conduction and modulate a range of cellular responses largely via G-protein–coupled receptors (GPCRs). In nonmammalians, efferent systems from the CNS target ACs with fast neurotransmitter synapses, especially GABA. In mammals, all known efferents appear to release either histamine or serotonin, likely as volume signaling systems.
HC noncanonical signaling
HCs generate potent large-field, slow surround signals in retinal RGCs and BCs, and even in nonmammalian cone photoreceptors. There is evidence for feedforward signaling via the cone → HC → BC path, feedback signaling via the cone → HC → cone → BC path, , and, now, the rod → HC → rod. The efficacy and sustained nature of the feedback signal is such that no known vesicular mechanism could maintain it (other than a ribbon-style synapse). Vesicular HC synapses are very rare and small. Several models of noncanonical signaling have been proposed, including synaptic pH regulation, hemijunction-mediated ephaptic signaling, and even transporter-mediated signaling. Some of these will be discussed in detail further in the chapter, but this unusual functionality is further evidence that HCs are not classical neurons.
Coupling types and coupling patterns
Although gap junctional coupling was first discovered between HCs, only in the last decade has it become clear that intercellular coupling is pervasive in retina. There are two simple classes of coupling: homocellular and heterocellular (between like and different classes of cells). The participant connexins in each case are likely to be homotypic or heterotypic (similar or dissimilar connexin types). The strength of coupling is associated with the size of the junctions, as they represent summed parallel conductances, and with functional modulation by various signaling pathways. Activated dopamine D1 receptors decrease conductances between coupled HCs , and coupled ACs, and dopamine D2 receptors modulate rod-cone coupling. The significance of coupling is clear in certain cases, such as the ability of HCs to spatially integrate signals over large fields (>1 mm diameter) or the crossover of rod signals into cones via heterocellular rod-cone and rod AC–cone BC coupling. Recently, heterocellular coupling between ACs and RGCs (AC::RGC) has been analyzed by ultrastructural connectomics and reveals that the most common forms involve OFF AC::OFF RGC and ON AC::ON RGC. Extensive coupling occurs among sets of cone BCs in which each class of BCs displays a unique set of partners.
Fast focal neurochemistry, synaptic currents, and amplification
One of the most powerful discoveries of the last two decades has been the diversity of the primary fast neurotransmitter receptors of the vertebrate nervous system. Again, the primary signaling channel of retina is the vertical glutamatergic chain from photoreceptors to brain. Rods, cones, and BCs encode their voltage responses as time-varying glutamate release. The targets of photoreceptors and BCs, in turn, decode time-varying extracellular glutamate levels as time-varying currents with glutamate receptors. There are two major classes of glutamate receptors: ionotropic and metabotropic (iGluRs and mGluRs, respectively). The iGluRs are separable into two distinct families: the AMPA/KA receptors and NMDA receptors. AMPA and KA receptors are related but pharmacologically and compositionally distinct. Four basic classes of glutamate receptor subunits (GluR1, 2, 3, 4) can be recruited to form a tetrameric AMPA receptor. Similarly, five basic classes of KA receptors (GluR5, 6, 7, and KA1, 2) can be assembled into tetrameric KA receptors. With some exceptions, these receptor assemblies can have nearly any stoichiometry. NMDA receptors are a distinct group of iGluRs in several ways. First, they have an obligate tetrameric subunit composition. Second, they are coincidence detectors that require dual activations by glutamate and by a glycine-like endogenous agonist. There is substantial evidence that this co-ligand may be D-serine released from Müller glia. Finally, the mGluRs represent a complex collection of GPCRs whose functions are far from clear.
Different classes of neurons express different types or different combinations of receptors and, in the end, the glutamate receptor profile of a cell appears diagnostic for its class. Mammalian BCs are unique in expressing either mGluR6, KA, or AMPA receptors as their glutamate decoding system. But immunochemical and mRNA expression analysis suggest that these associations are not so precise, as iGluR subunit expression occurs in nominally mGluR6-driven cells. HCs predominantly express AMPA receptors but show no NMDA-mediated responses. Finally, ACs and RGCs resemble CNS neurons in expressing AMPA receptors augmented by varying amounts of NMDA receptors.
The key glutamate receptor systems of retina operate on the principle of cation permeation. When activated, iGluRs generate increased channel conductances and carry inward currents carried mostly by Na + and Ca 2+ . Thus the canonical iGluR AMPA, KA, and NMDA families of receptors are nominally sign-conserving (>) depolarizing systems that “copy” the polarity of the presynaptic source voltage input in the postsynaptic target. The facts that many inputs converge on one postsynaptic cell; that small presynaptic voltages can modulate the release of many vesicles (in SNE cells); and that glutamate gates large postsynaptic conductance changes to cations with a positive reversal potential means that such synapses have high gain. Signals from photoreceptor to brain are successively amplified by a chain of glutamate synapses.
The group III mGluR6 system is unique and in retina is expressed by ON BCs ( Box 21.2 ). No known multipolar neuron in the CNS uses this receptor as its primary signaling modality. As a classical GPCR, with Goα as its cognate G-protein, the binding of glutamate triggers a cascade of signals that leads ultimately to the closure of cation channels on BC dendrites, thus moving the BC membrane potential closer to the K + equilibrium potential. Thus mGluR6 receptors are nominally sign-inverting (>i) hyperpolarizing systems that invert the polarity of the input in the postsynaptic target. The modulation of a strong cation current renders the mGluR6 mechanism high gain in spite of its inverted polarity.
Glutamate excitotoxicity has been invoked as a mechanism in retinal diseases. The evidence is mixed and controversial. On balance:
- •
Elevations of vitreal glutamate in glaucoma have not been validated.
- •
Glaucoma-mediated loss of retinal ganglion cells (RGCs) does not match known excitotoxic patterns.
- •
Starburst amacrine cells (Acs) are the most glutamate-sensitive cells in the retina but there are no established AC losses in primate glaucoma.
- •
NMDA receptor antagonists appear neuroprotective in ocular hypertension models of glaucoma but the mechanism of NMDA neuroprotection may be indirect:
- •
Retinal RGC death in glaucoma likely involves Ca +2 -mediated apoptosis.
- •
NMDA antagonists (like many drugs) will decrease Ca +2 loads in neurons.
- •
Weak NMDA antagonists likely have no lasting role in glaucoma therapeutics.
- •
- •
Glutamate is likely a major player in retinal damage in diabetes and ischemic insults.
- •
Neuroprotection is difficult to achieve in those cases as it involves AMPA receptors.
- •
Excitoxicity in hypoxic retina is likely initiated by reverse transport of glutamate by BCs.
- •
Competitive, nontranslocated transporter ligands may be safer ocular neuroprotectants.
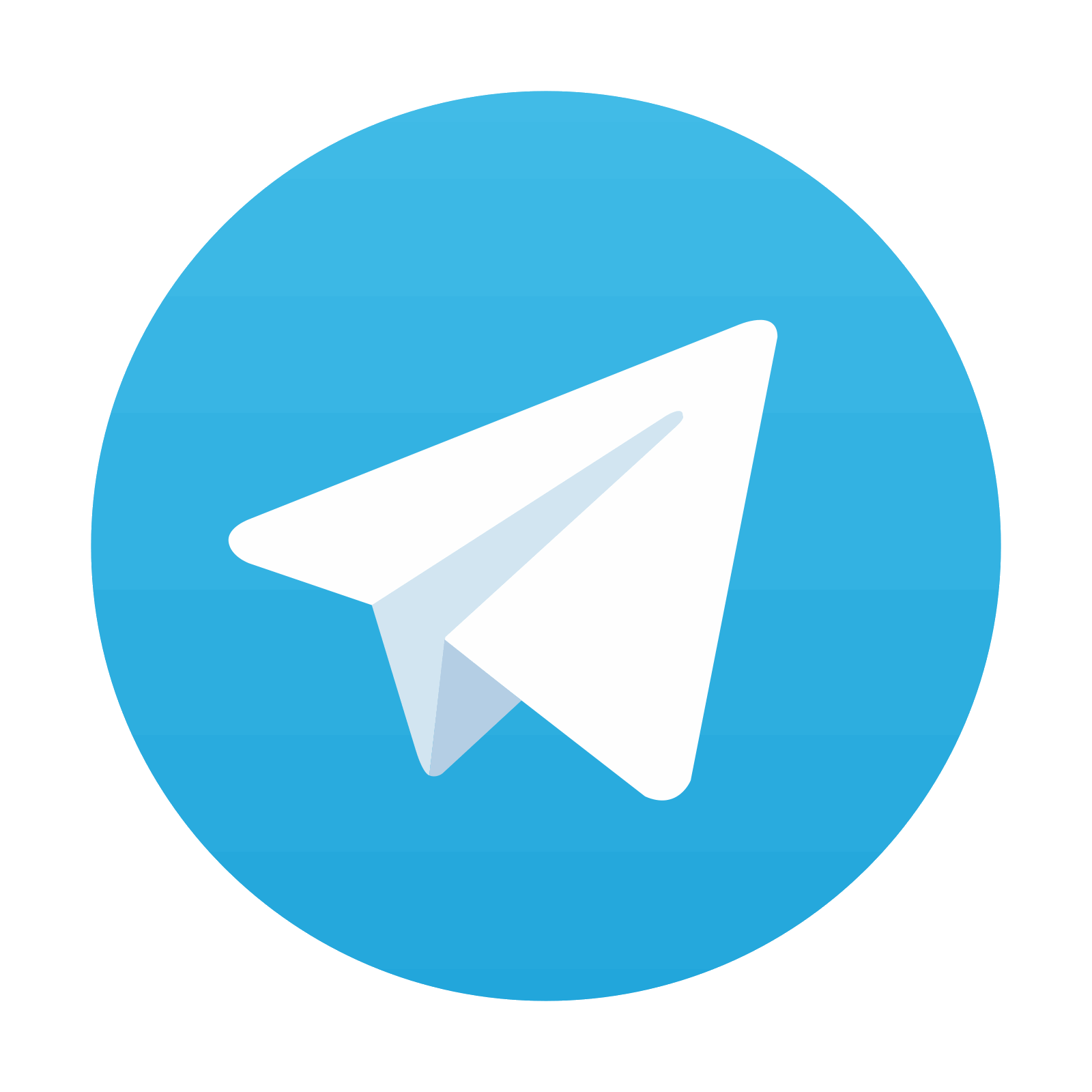
Stay updated, free articles. Join our Telegram channel
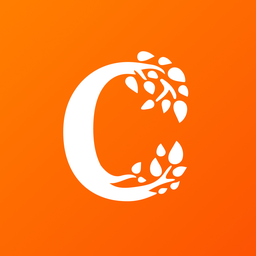
Full access? Get Clinical Tree
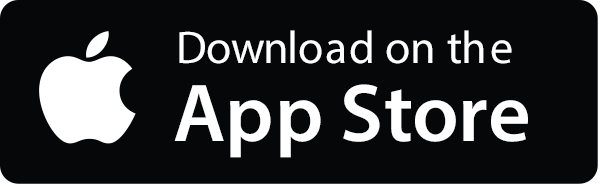
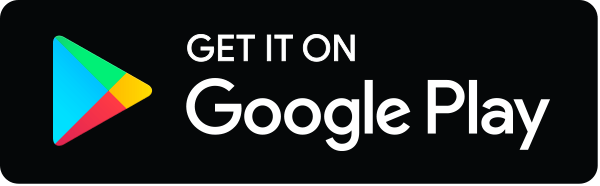
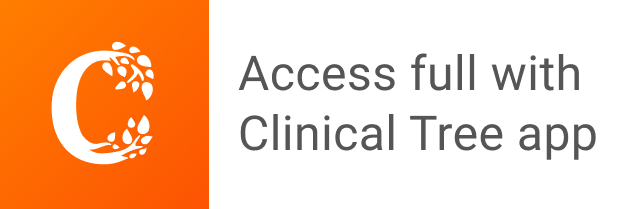