Introduction
The optical properties of the ocular lens are a critical determinant of overall vision quality. As a transparent specialized epithelial tissue, the lens contributes to the overall and dynamic focusing power of the eye and corrects for optical errors introduced by the cornea. To perform these functions, the lens needs not only to be transparent to avoid light scattering but also to have a higher refractive index than the medium in which it is suspended. It also needs to have refractive surfaces with the appropriate curvature to ensure that light is correctly focused on the retina. Furthermore, to compensate for its own spherical aberration (see Box 5.1 ) and that of the cornea, the lens generates a gradient of refractive index (GRI) that improves overall vision quality. Thus, the critical tissue level parameters that determine the focusing power of the lens are its surface curvature (geometry) and GRI. However, as might be expected of a living tissue, these parameters change as we grow. Therefore the transparent (light scattering) and the refractive (optical power and dynamic focusing) properties of the lens are not constant and change throughout life. Some of these changes are compensated for by alterations to lens power to ensure that the focal point and vision quality of the eye remains remarkably constant despite the continual growth of the lens. However, other age-dependent changes to the lens reduce the dynamic focusing or accommodative ability of the lens that manifests as presbyopia in middle age, while increased light scattering produces a loss of transparency that leads to cataract formation in the elderly. Together these two lens pathologies are by far the leading causes of uncorrected refractive error and blindness, respectively, in the world today.

Spherical aberration is an example of a subtle optical imperfection or higher-order aberration that results in a loss of clarity of images formed by the eye and is more complex than simple refractive errors such as astigmatism, near-sightedness, and far-sightedness. Spherical aberration is a specific type of optical aberration. It occurs when light rays passing through the periphery of the cornea and lens come to a focus at a slightly different location than light rays passing through the center of the cornea and lens. This results in decreased image quality. Spherical aberration tends to increase with pupil size and is therefore more noticeable in low light conditions than in bright sunlight. Visually, spherical aberration causes blur, loss of contrast, and halos around lights.
At the molecular and cellular levels, the transparency and refractive properties of the lens are established by a specialized tissue architecture that is first established in utero, and which is maintained throughout life by a unique physiology. This chapter provides an overview of how lens development, growth, metabolism, and physiology establish and maintain the optical properties of the normal lens. It also explores how age-related changes in these processes contribute to the observed change in lens power that occurs with age, the onset of presbyopia in middle age, and the formation of different forms of cataract in the elderly. Information about the human lens is emphasized, although studies using animals are included when human data are unavailable. The unsolved issues in the biology and pathology of the lens are highlighted throughout. References to comprehensive reviews and original source material are included to assist the reader. The citations provided are not meant to be inclusive, but to highlight articles of special relevance.
The organization, development, and growth of the lens
Because the lens grows throughout life and consequently changes its geometry and therefore its optical power, we will initiate our discussion of the organization of the lens by considering the lens from a typical adult subject that can accommodate. At this stage of life, the unaccommodated lens of a young adult typically exhibits a flattened oblate spheroid shape with a diameter of approximately 9 mm, a thickness of approximately 4.5 mm, and anterior and posterior surface radii of curvature of some 10 mm and 5.5 mm, respectively. This lens contributes around 20 diopters (D) of optical power to the emmetropic eye, corrects for corneal spherical aberration, and can accommodate. It is located in the eye behind the iris, and its position in the optical pathway is maintained by the zonules of Zinn that attach the lens to the ciliary body ( Fig. 5.1 ). During the process of accommodation, contraction of the ciliary muscle causes the ciliary attachments of these zonular fibers to move forward and inward, thereby allowing the elastic, collagen-rich capsule that surrounds the lens to mold its shape. Thus, in our typical young adult, the accommodated lens assumes a rounder shape and increases its optical power by approximately 10 to 12 D to enable focusing on near objects. The cellular organization of this typical young adult lens will be first considered before discussing the processes during embryonic development and growth that establish the structural features of the adult lens.

Cellular organization of the adult human lens
The zonules that suspend the lens within the eye originate in the nonpigmented layer of the ciliary epithelium and insert into the lens capsule that surrounds the lens near the equator ( Fig. 5.2 ). Examination of the insertion of zonular fibers into the lens capsule that surrounds the lens shows that these fibers are intimately interwoven with the components of the collagenous lens capsule. The extracellular matrix that forms the lens capsule is continuously secreted by epithelial and superficial fiber cells. , It is composed predominantly of type IV collagen, laminin, entactin (nidogen), and the heparan sulfate proteoglycan perlecan. The capsule is composed of multiple laminae, as if the basal lamina had been replicated many times, and the different laminae appear to have distinctive compositions in different regions. At the lens equator, collagen IV and nidogen span the capsule depth, while laminin and perlecan are located in two separate lamellae located at the innermost and outermost capsule domains. The lamina structure of the capsule suggest that it is synthesized from the inside out. This contention has been supported by studies of the synthesis of the lens capsule in experimental animals. These have shown that newly synthesized capsular materials are originally deposited close to the basal ends of epithelial and fiber cells, but over time move farther away from the surface of the cells as they are displaced by successive layers of newly synthesized capsular material. These observations suggest that the rate of synthesis of components of the capsule at its inner surface and the rate of degradation at its outer surface regulate capsule thickness. The enzymes that are responsible for the degradation or remodeling of the capsule have not been identified.

The rest of the lens is formed from two populations of cells. A sheet of cuboidal cells, the lens epithelium, covers the surface of the lens closest to the cornea, while the bulk of the lens consists of concentric layers of elongated fiber cells that are at different stages of differentiation ( Fig. 5.3 ). The outer shells of fiber cells extend from just beneath the anterior epithelium to the posterior lens surface, a distance of over 1 cm in adults. In the adult lens, most epithelial cells and all fiber cells are nondividing. Only cells near the equatorial margin of the lens epithelium, in a region called the germinative zone, undergo a slow proliferation ( Fig. 5.3A,B ). Most of the cells produced by mitosis in this region migrate toward the posterior of the lens and differentiate into fiber cells at the lens equator. These new differentiating fiber cells undergo extensive elongation and express a number of fiber-specific proteins. It needs to be remembered, however, that fiber cells are essentially elongated epithelial cells that retain their distinct apical and basal membrane domains but have dramatically elongated lateral membranes ( Fig. 5.3C ). To adopt the hexagonal cross-sectional shape used by fiber cells to achieve an orderly packing that minimizes their extracellular space, lateral membranes of fiber cells are further subdivided into distinct broad- and narrow-side membrane domains ( Fig. 5.3D ).

During elongation, the posterior (basal) ends of the fiber cells migrate along the inner surface of the capsule, and their anterior (apical) ends migrate beneath the epithelium until they meet elongating cells from the other side of the lens near the posterior and anterior midlines to form the lens sutures. In some species, all fiber cells meet near the midline of the lens, forming an “umbilical” suture. In most species, the sutures form along planes. In human embryos, elongated lens fiber cells meet at three planes, forming an upright “Y” at their anterior ends (with respect to the superior-inferior axis of the eye) and an inverted “Y” posteriorly ( Fig. 5.4A ). As the human lens grows, the suture planes formed by more superficial shells of fibers become increasingly complex. The first evidence of this typically occurs soon after birth, when two new suture planes form at the ends of each of the three branches of the Y sutures ( Fig. 5.4B ). As new fibers are added during lens growth, the branch points of the newly formed sutures gradually “migrate” toward the center, eventually forming a six-pointed “star” suture ( Fig. 5.4C ). Branching again occurs at the tips of each of these six planes, eventually forming a total of 12 suture planes at the anterior and posterior surfaces of the lens ( Fig. 5.4D,E ). The increasing geometric complexity of the suture patterns in older human lenses results in lenses with better optical properties than in species that maintain a simple Y suture pattern throughout life.

Once fiber cells reach the sutures, they stop elongating and their basal ends detach from the capsule. Soon after reaching the sutures, fiber cells degrade all intracellular membrane-bound organelles, including their nuclei, mitochondria, and endoplasmic reticulum. Organelle-free mature fiber cells are gradually buried deeper in the lens as successive generations of fibers elongate and differentiate. In this way, the lens continues to increase in size and cell number throughout life. Because protein synthesis ceases just before organelle degradation, the components of mature fiber cells must be much more stable than those in cells found in other parts of the body. In fact, since the fiber cells in the center of the lens are present at birth and persist until death (or cataract surgery), their constituent proteins and membranes may last for more than 100 years. Because this process occurs throughout life, a gradient of fiber cell age is established, with the oldest mature fiber cells in the lens nucleus having been laid down during embryogenesis.
Development of the embryonic lens
The adult lens is formed by cells that were originally part of the surface ectoderm covering the head of the embryo. Interactions between the future lens cells and nearby tissues during early development give these cells a “lens forming bias.” As a result of these interactions, patches of cells that lie on either side of the head are marked by expression of the transcription factor, Pax6, which is a master regulator of lens and eye development. At the same time, neural epithelial cells on either side of the diencephalon in the embryonic forebrain bulge laterally to form the optic vesicles, which eventually contact the surface ectoderm cells ( Fig. 5.5A ). The cells of the optic vesicle also express Pax6, and Pax6 function in these cells is essential for eye formation. Many of the genes that regulate and are regulated by Pax6 during the early stages of eye formation are now known and have been extensively reviewed. Hence, in this section we will concentrate only on the morphologic changes that occur during embryonic development to first establish the structure of the lens that is maintained throughout life.

After they make contact, the optic vesicles and the prospective lens ectoderm cells secrete an extracellular matrix that causes these cell layers to adhere tightly to each other ( Fig. 5.5B ). The surface epithelial cells then elongate, forming the thickened lens placode ( Fig. 5.5B ). Soon afterward, the lens placode and the adjacent cells of the optic vesicle buckle inward to form the lens pit ( Fig. 5.5C ). This morphologic transformation is accompanied by the formation of the bilayered optic cup. The invaginated lens placode soon separates from the surface ectoderm by a process involving the death of the cells in the connecting stalk between the cells of the surface ectoderm and the lens ( Fig. 5.5D ). The Pax6-expressing ectodermal cells adjacent to the lens placode that remain on the surface of the eye become the corneal and conjunctival epithelium. During lens invagination, the extracellular matrix between the optic vesicle and the lens begins to diminish and the two tissues separate ( Fig. 5.5E ). The space that is formed between them is rapidly filled with a loose extracellular matrix, the primary vitreous body, that is secreted by the cells of the inner layer of the optic cup ( Fig. 5.5E ).
The epithelial cells that give rise to the lens vesicle originally lie on a thin basal lamina. During the process of invagination, this basal lamina surrounds the lens vesicle. It gradually thickens by the deposition of successive layers of basal lamina material to form the lens capsule. Soon after the lens vesicle separates from the surface ectoderm, the cells in the portion of the vesicle that are closest to the retina begin to elongate. The elongation of these primary fiber cells soon obliterates the lumen of the vesicle as their apical ends contact the apical ends of the anterior epithelial cells ( Fig. 5.5E,F ). Primary fiber cell formation establishes the fundamental structure of the lens, with epithelial cells covering the anterior surface and elongated fiber cells filling the bulk of the lens.
At early stages of lens formation, most of the lens epithelial cells are actively proliferating. Cells at the margin of the epithelium are displaced posteriorly and migrate toward the equator, where they are stimulated to differentiate into secondary fiber cells by growth factors present in the vitreous body. As the secondary fibers elongate and their basal and apical ends migrate toward the poles of the lens, they displace the central primary fibers from their attachments with the capsule and the lens epithelium. This process buries the primary fiber cells in the center of the lens to form the embryonic nucleus ( Fig. 5.3A ).
Because this process of secondary fiber cell differentiation and internalization of existing cells occurs throughout life, the lens increases in size over time with either a monophasic or biphasic growth rate that is species specific. For most species, lens growth is monophasic, being characterized by a period of rapid growth during early development that slows postnatally to approach an asymptotic maximum by the end of the life span. In contrast, the human lens exhibits a biphasic growth rate in which lens growth in utero and in the immediate postnatal period is asymptotic, but thereafter the lens grows linearly (but slowly) at a rate of about 1.38 mg/year. This generates two distinct compartments, the prenatal and the postnatal. The prenatal growth mode leads to the formation of an adult nuclear core of fixed dimensions and the postnatal to an ever-expanding cortex. The nuclear core and the cortex have different properties and can readily be physically separated. In addition, the composition (dry matter to wet matter) of the lens tends to change over time as the lens grows. By simply weighing lenses of different ages before and after drying, the rate of increase in dry weight compared with the increase in wet weight can be calculated. This analysis showed that the proportion of dry weight increases significantly with age in many species. This phenomenon is believed to reflect the time-dependent compaction of fiber cells in the lens interior, suggesting that this process is driven by the removal of water from the cytosol of the inner fibers, leading to elevated protein concentrations. This in turn contributes to the development of the GRI.
In most species, lens shape appears to scale with growth, in that its aspect ratio (sagittal thickness/equatorial diameter) remains relatively constant across the life span. However, consistent with its biphasic growth pattern, the shape of human lens does not simply scale with growth ( Fig. 5.6 ). Early in embryonic development, the human lens is almost spherical ( Fig. 5.6A ) and remains that way until shortly after birth when, as part of the emmetropization process, it becomes increasingly elliptical, eventually losing approximately 20 D of refractive power by adulthood ( Fig. 5.6B ). This shape change is the result of an increase in the equatorial diameter and, remarkably, a decrease in sagittal thickness ( Fig. 5.6C ), which suggests the changes in the shape of the human lens during childhood and puberty reflect both compaction and remodeling of fiber cells in the lens interior. Then, during adulthood (>20 years of age), the continued growth of the lens produces similar increases in both the sagittal thickness and equatorial diameter ( Fig. 5.6D ) such that the aspect ratio remains fairly constant throughout adulthood.

Differentiation of secondary fiber cells
At the edge of the epithelium, near the lens equator, epithelial cells differentiate continuously into fiber cells ( Fig. 5.3 A,B ). In the germinative zone (GZ) of the epithelium, daughter cells produced by mitosis are eventually displaced (or migrate) into the transition zone (TZ). Cells in this TZ are postmitotic and have not yet begun to elongate, but as they move posteriorly, they are exposed to growth factors contained in the vitreous, which appear to be secreted from the retina, that stimulate their elongation. Several growth factors are capable of initiating lens fiber cell differentiation when added to cultured lens epithelia or, in some cases, when overexpressed in the lens in vivo. Among these are members of the fibroblast growth factor (FGF) and insulin-like growth factor (IGF) families. Studies using mouse lenses deficient in three of the four FGF receptors have conclusively demonstrated that FGF signaling is required for the formation of lens fiber cells. In contrast, other soluble factors, especially members of the bone morphogenic protein (BMP) family, appear to modulate or potentiate the effects of FGFs on lens fiber cell differentiation. This work has led to the development of the lens gradient hypothesis ( Fig. 5.7A ), in which the vitreous contains higher levels of FGF and associated enhancers of FGF signaling than the aqueous. However, there are other considerations, including the presence of recently identified growth factor signaling inhibitors, Sef, Sprouty, and Spreds, in the epithelium. Thus, while the FGF gradient hypothesis provides a good explanation for the initiation of the process of fiber differentiation, questions remain around the interaction of FGF with other growth factors and signaling pathways that sustain fiber cell differentiation so that the correct three-dimensional cell architecture of the lens is obtained.

In this regard, several additional signal transduction pathways are activated at different times during fiber cell differentiation and maturation to ensure that fiber cells elongate with the correct curvature, lose their organelles, and change their junctional morphology and hence cellular shape—all changes required to establish the optical properties of the lens. One such pathway that appears to coordinate the extension of the fiber cells is the planar cell polarity (PCP) mechanism that involves Wnt/Frz signaling. Differentiating fiber cells contain a cilium/centrosome that is polarized toward the side that faces the anterior pole ( Fig. 5.7B ), and depletion of multiple protein components of the PCP pathway disrupt cilium orientation and fiber cell morphology, which alters the surface curvature and hence optical properties of the lens. Cadherins, integrins, Rho-GTP proteins, and tropomodulin are all involved in the regulation of the lens cell cytoskeleton, the reorganization of which is associated with the change in cell morphology that occurs as fiber cells differentiate and elongate. However, the degradation of fiber cell nuclei, mitochondria, and other subcellular organelles appears to be driven by autophagy and mitophagy, with the inhibition of full apoptosis in fiber cells regulated by the IGF-1R/NF-κB pathway and β1-integrin signaling. More recently, Eph-ephrin signaling has been associated with cataract formation. The binding of Eph receptor tyrosine kinases to ephrin ligands leads to a bidirectional signaling pathway that controls many cellular processes. In the lens, EphA2 and ephrin-A5 have been shown to be involved in determining fiber cell shape, organization and patterning, as well as the overall optical and biomechanical properties of the whole lens. In summary, it appears that a variety of signaling pathways interact to coordinate the differentiation and maturation of fiber cells to order, to establish a level of cellular organization that results in the correct optical properties at the whole tissue level.
Structural determinants of the transparent and refractive properties of the lens
The transparent properties of the lens are the result of several structural adaptations that occur at the molecular and cellular levels in the different regions of the lens, all of which are designed to minimize light scatter. These adaptations include the loss of the lens-associated vasculature, the elimination of light-scattering intracellular organelles, an ordered cellular architecture in the peripheral lens, changes to fiber cell morphology to form a barrier to extracellular diffusion, and the matching of the refractive index of the membranes and cytoplasm of fiber cells in the lens nucleus. These cellular level adaptations also manifest as changes to the refractive properties of the lens at the whole tissue level that alter the geometry and the GRI, the two key parameters that determine the focusing power of the lens.
Loss of the lens vasculature
Soon after its formation, the embryonic lens becomes covered with a meshwork of capillaries. The portion of this network encasing the posterior lens, the tunica vasculosa lentis, arises from the hyaloid artery. The capillaries found at the anterior side of the lens, the anterior pupillary membrane, arise from blood vessels of the developing iris stroma. These capillary networks join with each other near the lens equator. It has been assumed the fetal vasculature is important for normal lens development, although similar vessels are never present around the lenses of nonmammalian species. Deletion of vascular endothelial growth factor (VEGF-A) from the lens prevented the formation of the fetal vasculature, resulting in smaller lenses with transient nuclear cataracts. Since mouse lens cells express functional VEGF receptors, further studies are required to determine whether these cataracts were due to loss of VEGF signaling in lens cells, the absence of the fetal vasculature, or both.
During the second trimester of human development, the capillaries of the tunica vasculosa lentis and the anterior pupillary membrane regress. Hence, by the fetal period, the lens is devoid of a blood supply, and therefore the avascular lens relies on the aqueous and vitreous humor for nutrients. Decreasing levels of plasma-derived VEGF may be one of the factors involved in the normal regression of these vessels. Macrophages in the vitreous body also appear to play an essential role in capillary regression. In mice, these macrophages cause the programmed death of the endothelial cells by secreting the morphogen, Wnt7b. A number of hereditary and acquired ocular diseases are accompanied by persistence of the fetal vasculature (see review ). At present, it is not clear why the fetal vasculature fails to regress in so many different syndromes and hereditary diseases. Better understanding of the factors that regulate vascular regression in normal ocular development is needed to address this question. However, regardless of the exact molecular mechanisms, loss of lens-associated vasculature is essential to establish normal vision by ensuring that light is not absorbed by heme pigments within the visual axis.
Loss of nuclei and cellular organelles
The degree to which lens organelles scatter light depends largely on the difference in refractive index between the organelle and the surrounding cytoplasm. However, as the refractive index of the cytoplasm is not matched to that of the organelles, the lens possesses a coordinated strategy for degrading organelles throughout the course of lens fiber cell differentiation, such that the majority of the fiber cells situated in the light path completely lack organelles. Soon after elongating, fiber cells detach from the posterior capsule and suddenly degrade all of their membrane-bound organelles, including their mitochondria, endoplasmic reticulum, and nuclei. This process of organelle degradation occurs very rapidly, being completed within a few hours. The loss of cell organelles from differentiating fiber cells produces a central organelle-free zone (OFZ) that consists of mature fiber cells that are incapable of protein synthesis or turnover of existing proteins, so that they are maintained throughout life. Disruptions to the formation of the OFZ are accompanied by the persistence of organelles in lens fiber cells, which in animal models have been shown to increase light scattering and contribute to cataract formation. In adult primates, the outer shell of fiber cells that contain organelles is only about 100 microns wide. These organelle-containing cells are located, for the most part, outside of the optical axis of the lens. Thus, the programmed removal of organelles in mature fibers enhances lens transparency by eliminating potential light-scattering elements. However, organelle removal also renders mature fiber cells in the center of the lens incapable of de novo protein synthesis.
The mechanisms underlying the process in which organelle systems are rapidly degraded initially appeared similar to apoptosis (programmed cell death), since chromatin condensation and marginalization occur, as well as DNA fragmentation between nucleosomes. However, unlike apoptosis, the cytoskeleton is maintained in lens fiber cells, even though organelles have fully disappeared. This indicates that the underlying mechanisms that control organelle loss in differentiating lens fiber cells, relative to cells undergoing apoptosis, are different. Indeed, in recent years it has emerged that the process of organelle degradation has more in common with the processes of autophagy and mitophagy, which are designed to ensure cell survival, rather than the cellular mechanisms that drive apoptotic cell death.
Autophagy is a cellular process whereby damaged or excess organelles and macromolecules are degraded in a stepwise program. Originally considered a specific response to cellular starvation or stress, it is now recognized that autophagy plays a major part in the regulation of cellular development and differentiation, as well as the ongoing maintenance of cellular homeostasis. Classic autophagy begins with the formation of a phagophore, a membrane that surrounds cellular materials or organelles targeted for degradation. Phagophores expand to become autophagosomes, which then fuse with lysosomes to form autophagolysosomes or autolysosomes where final degradation of the contents takes place. A plethora of signaling and regulatory molecules are required not just to initiate phagophore formation and complete autophagy but also to target specific cellular organelles/macromolecules for degradation. Indeed, it appears that each organelle has a specific autophagy pathway for its degradation, with mitophagy the selective autophagy process in which mitochondria are degraded. Hence, the elucidation of the complex repertoire of pathways and events leading to lens organelle elimination is an ongoing area of research. current results suggest, however, that distinct but coordinated mechanisms function to regulate the elimination of the nucleus, mitochondria, endoplasmic reticulum, and Golgi apparatus that occurs during fiber cell differentiation so that these light-scattering elements are removed from the optical pathway in the deeper lens.
Ordered cellular architecture of lens fiber cells
It has long been thought that, in addition to the removal of light-scattering elements, the ordered cellular architecture of the “crystalline” lens is a major contributor to lens transparency. However, this ordered cellular structure is only really evident in differentiating fiber cells in the outer cortex, and as fiber cells undergo further differentiation and become internalized deeper into the lens, they exhibit changes to their morphology that disrupt this initial cellular order ( Fig. 5.8 ). Hence, the relative contribution of fiber cell morphology to lens transparency changes between the cortex and the nucleus. These regional differences in fiber cell morphology are thought to be driven by age-dependent changes to the cytoskeletal and junctional proteins that modulate fiber morphology. The distinctive hexagonal fiber cell morphology found in the outer cortex is the net result of the interaction of a variety of junctional and cytoskeletal proteins, and this complement of proteins changes as fiber cells differentiate and become internalized within the lens. In differentiating fiber cells, these changes in protein expression can be initially achieved via de novo protein synthesis of a specific complement of proteins required at that specific stage of the differentiation process. However, once a fiber cell enters the OFZ and loses its ability to synthesize new proteins, any further changes in cellular components required to elicit the observed changes in fiber cell morphology and function in the OFZ needs to be driven by post-translational modifications to the existing pool of lens proteins.

Differentiation-dependent changes to membrane junctions
Lens cells express many of the proteins that form the junctions (i.e., adherens junctions and gap junctions) typically found in other cell types. The lens also contains a number of unique membrane specializations that are comprised of distinct junctional complexes that contribute to the distinctive morphology of lens fiber cells. Because fiber cell morphology changes from the outer cortex to deeper nucleus, so does the complement of cell junctions and membrane specializations ( Table 5.1 ). In the lens cortex, adherens junctions, immunoglobulin superfamily (IgSF) proteins, and gap junctions are the predominant junction-forming proteins. However, as fiber cells differentiate and become internalized, adherens junctions disappear, the morphology of gap junction plaques changes, ball-and-sockets are less prevalent, and tongue-and-groove membrane interdigitations and membrane fusions become the dominant features in mature fiber cells in the lens nucleus.
Lens region | Fiber cell junctional feature | Main associated protein(s) | Cell membrane localization | Proposed function |
---|---|---|---|---|
Cortex | Cortex adhaerens | N-cadherin, α-/β-catenin, plakoglobin, p120ctn, vinculin | Narrow side | Cell adhesion, actin cytoskeleton linking, intermediate and beaded filament linking |
Ezrin, periplakin, periaxin, desmoyokin | Broad side | Cell adhesion, actin cytoskeleton linking, intermediate and beaded filament linking | ||
Gap junction | Cx46, Cx50 | Broad side | Cell adhesion, cell communication | |
Immunoglobulin Superfamily adhesion proteins | Nr-Cam, Cadm1, N-Cam | All | Cell adhesion | |
Edge protrusions | Cell vertices | Cell interlocking | ||
Ball-and-sockets | Cx46, Cx50 | Broad side | Cell interlocking | |
Nucleus | Gap junction | Cx46 (C-terminally truncated), Cx50 (C-terminally truncated) | All | Cell adhesion, cell communication |
Tongue-and-grooves (microplicae) | AQP0 (C-terminally truncated) | All | Cell interlocking | |
Square arrays | AQP0 (C-terminally truncated) | All | Cell adhesion | |
Compaction folds | All, orthogonal to fiber cell long axis | Cell shortening | ||
Cell fusions | MP20 | All | Cell communication |
Differentiation-dependent changes to the composition and ultrastructure of fiber cells junctions
The adherens junctions found between cortical fiber cells are composed of the calcium-dependent cadherin proteins that complex with catenin proteins to link the actin cytoskeleton of neighboring cells together. N-cadherin is the most prominent cadherin present in lens fiber cells. Previously, it has been proposed that the large lateral fiber cell domains represent a tissue-specific adherens junctional structure, termed the cortex adhaerens . In this structure, cytoskeletal interacting proteins are localized to narrow- or broad-side membranes to maintain cortical fiber cell structure. Also present in cortical fibers are gap junctions, which contain connexin (Cx) protein isoforms and form not only cell-to-cell adhesions but communicating channels between adjacent cells. Cx46 and Cx50 are abundant in lens fiber cells, and are found in large plaques on the broad sides of differentiating fiber cells in the outer cortex. IgSF proteins that facilitate calcium-independent adhesion are also present in the membranes of elongating fiber cells.
Cortical fiber cell membrane interdigitations appear as cellular projections along the vertices of the large lateral membrane domain ( Fig. 5.9A,B ). These edge protrusions are regularly arrayed along the length of fibers and fit into complementary-shaped pockets formed between neighboring lens fiber cells. It is speculated that these structures, which are present in lens fiber cells of all ages, physically interlock cells and may be important to resist the shear forces that are generated during lens accommodation. Smaller membrane interdigitations are also found emanating from broad face surfaces in a random pattern. These are characterized by cellular outpocketings, or balls, that consist of a globular portion attached by a short cylindrical cytoplasmic stalk and fit into complementary sockets in fiber cells of neighboring growth rings ( Fig. 5.9C,D ). Ball-and-socket joints are thought to play a role in the interlocking of fiber cells to minimize extracellular space and therefore light scattering.

Mature secondary lens fiber cells exhibit a markedly different morphology and complement of cell junctions and membrane specializations in comparison to cortical fibers. Adherens junctions are generally absent, probably owing to the degradation of the N-cadherin protein in lens development and aging. In addition, the large broad-side gap junction plaques have fragmented and instead are distributed throughout the cell membrane. In addition to the breakup and dispersal of gap junction plaques, ball-and-sockets are less prevalent in the highly compacted lens nucleus, while other morphologically distinct membrane structures are present. The tongue-and-groove membrane interdigitations ( Fig. 5.9E,F ), also known as microplicae, are frequent membrane ridges that form close membrane appositions, and are prominent in the lens nucleus. Tongue-and-groove interdigitations cover the surface of the nuclear fibers and stain asymmetrically for AQP0, the most abundant lens integral membrane protein. Square arrays that are a feature of the tongue-and-groove interdigitations are thought to contain AQP0 and/or its cleavage products. The morphology of these arrays suggests a role for AQP0 in cell-to-cell adhesion. These observations suggest that post-translational changes to junctional proteins in different regions of the lens alter not only the morphology of individual fiber cells but also the intracellular and extracellular interactions between cells.
Effect of changes in fiber cell junctions on regional differences in lens structure
The changes in the composition and ultrastructure of membrane junctions described in the previous section serve to adapt fiber cell structure to establish the key structural features in the different regions of the lens required to maintain the transparent, optical, and biomechanical properties of the whole lens. As fiber cells differentiate, they change their classical hexagonal shape and exhibit several distinct features that alter the morphology and therefore the function of deeper fiber cells. Again, it should be remembered that many of these changes occur in mature fiber cells that are incapable of de novo protein synthesis and therefore involve the post-translational modification of existing proteins to modify their membrane localization, interaction with other proteins, and regulation.
Large gap junction plaques on the broad sides of cortical fiber cells undergo a process of fragmentation and dispersion as fiber cells differentiate, which has been shown to correlate with a change in the direction of the flow of solutes between cells. In peripheral fiber cells, the large broad-side plaques serve to preferentially direct solute flows within fiber cell columns toward the lens surface, while the subsequent fragmentation and dispersal of the plaques promotes a more isotropic flow of solutes between deeper mature fiber cells. In addition, the cleavage of the cytoplasmic tails of Cx46 and Cx50 alters the function and pH regulation of gap junction channels in the lens nucleus. These observations suggest that changes to the ultrastructural organization of the gap junctional plaques and the subsequent cleavage of the pH-sensitive C-terminal tails of Cx46 and Cx50 are post-translational modifications designed to modify the direction of solute flows within the lens, as well as to ensure that the normally pH-sensitive gap junctions can operate in the low pH environment established by anaerobic metabolism in the lens nucleus.
Fiber cell compaction is a morphologic feature that occurs in nuclear fiber cells that correlates with the observed loss of cell volume. Scheimpflug imaging suggests that fiber cell compaction is initiated in the lens nucleus but also occurs in the lens inner cortex, and is most rapid in young lenses. Accordion-like “compaction folds” oriented orthogonal to the long axis of primary fiber cells are evident in the embryonic nucleus, while inner cortical fiber cells display numerous tongue-and-groove or microplicae junctions that become increasingly numerous with depth and completely cover the surface of nuclear fibers. The close apposition of the plasma membranes of neighboring cells ensures that the topology of the microplicae on one cell is matched by a complementary series of folds on the neighboring cell. In the absence of cellular organelles, it appears the remodeling of the fiber cell membrane is driven by a reduction in cell volume that causes fiber cell membranes to become folded and compacted. This compaction of fiber cells means that despite the constant growth of the lens throughout life, the lens can still be fit within the eye that stops growing.
A barrier to extracellular diffusion has been observed in the inner cortex of lenses in a number of species. This extracellular diffusion barrier has been proposed to restrict the movement of solutes into the lens and acts to direct nutrients and antioxidants into the lens core via the suture at both poles. The formation of this barrier appears to be associated with significant membrane and cytoskeletal protein remodeling. At the ultrastructural level, a number of structures could be involved in this observed closure of the extracellular space. Previous electron microscopy data suggested that the reduction of lenticular intercellular space was correlated with the formation of square array and membrane undulations. Thus, square arrays might potentially drive the formation of complicated membrane interdigitations and serve to maintain an extremely narrow extracellular space. In addition, it has been reported that gap junction plaques on the broad side of the outer fiber cells restrict penetration of larger solutes through the extracellular space. More recently, spatial resolved proteomic analysis of the region of the lens where the extracellular barrier forms have shown that AQP0 and its interacting partners, ezrin and radixin, are upregulated in this barrier region. Because ezrin and radixin link AQP0 to the cytoskeleton, these data suggest that changes in the membrane distribution of AQP0 may play an important role in controlling the narrowing of the extracellular spaces between fiber cells.
Membrane fusions between adjacent fiber cells have been observed in the deeper regions of a variety of lenses. These membrane fusions have subsequently been shown to form a functional pathway for the diffusion of large proteins between mature fiber cells within discrete growth rings. Interestingly, in the mouse lens, the formation of this lens syncytium was dependent on the presence of the abundant integral membrane protein MP20. These fusions have implications for the establishment of the GRI (see further discussion in this chapter).
Differentiation-dependent changes to cytoskeletal proteins
The lens is a specialized tissue that is specifically reliant on the establishment of a precise cytoarchitecture to determine and maintain structure that, in turn, is critical for its function, transparency, and biomechanical properties. This structure is determined by cytoskeletal networks, including microtubules, intermediate filaments, and actin microfilaments, and their interactions with cell adhesion junctions and fiber cell–specific membrane specializations. These cytoskeletal networks also act synergistically to regulate lens structure and, consequently, function.
Microtubules : Microtubules are abundant beneath the plasma membranes of lens fiber cells where they probably play an important role in stabilizing the fiber cell membrane. Microtubules may also be important for transporting vesicles to the apical and basal ends of elongating fiber cells, although neither function has been demonstrated in vivo. Microtubules are poorly expressed in the lens epithelium and nuclear fiber cells but are localized in lengthwise arrays in the peripheral cytoplasm of cortical fiber cells, which suggests they may contribute to the processes associated with fiber cell elongation. As in other cells, microtubules in the lens also mediate vesicle trafficking, through kinesin and dynein motors, to both lens poles, which is likely important to establishing polarity during lens development. For example, they are known to transport vesicles harboring membrane proteins, such as aquaporin 0 (AQP), during fiber cell differentiation and elongation. They may also facilitate the formation of the OFZ, which is crucial for continued transparency. A mutation in a mediator of microtubule plus end-directed vesicle transport, FYVE And Coiled-Coil Domain Autophagy Adaptor 1 (FYCO1), causes a form of autosomal-recessive congenital cataract. As FYCO1 is known to associate with autophagosomes in other cells, microtubules may participate in autophagosome trafficking.
Recently it was noted that the stabilized (acetylated) population of microtubules, localized close to the epithelial–fiber cell interface, was specifically involved in fiber cell elongation, as loss of the dynamic microtubule population alone did not affect lens fiber cell elongation or the directionality of their migration. Interestingly, this function was mediated through the regulation of myosin 2 activation. Moreover, through their association with N-cadherin junctions, specifically in elongating fiber cells, stable microtubules regulate N-cadherin junction organization along the lateral borders. As these junctions are epicenters of actin organization, this interaction suggests that stable microtubules may impact actin organization during lens morphogenesis.
Actin: The actin cytoskeleton in the lens interacts closely with fiber cell membranes to maintain epithelial cell morphology and polarity, and to influence fiber cell differentiation. Complexes of F-actin and adherens junctions can be found at epithelial–epithelial, epithelial–fiber, and cortical fiber–fiber cell membrane contacts. Of the different actin networks, the spectrin-actin skeleton is the best characterized in the lens. It consists of actin filaments crosslinked by α 2 β 2 -spectrin, stabilized along their sides by γ-tropomyosin (γTM), and capped at their barbed and pointed ends by adducin and tropomodulin 1 (Tmod1), respectively. The meshwork is thought to be anchored at the fiber cell membrane via the interaction of spectrin with ankyrin-B, which, in turn, is linked to cadherin-based adherens junctions and the ezrin, periplakin, periaxin, and desmoyokin (EPPD) anchorage complex. The relationship between the N-cadherin junctions and actin cytoskeleton appears to be reciprocal in that the cytoskeleton determines cadherin junction maturation, while independent maturation of cadherin junctions also appears to drive organization of the cortical actin cytoskeleton and elongation of fiber cells.
Rearrangement of the basal network of actin (actin-rich lamellipodia and stress fibers) in the anterior epithelial cells, which tethers them to the lens capsule, is necessary to drive fiber cell elongation and differentiation, and ends in the formation of a terminal web that stabilizes the ends of fiber cells at the sutures. Upon differentiation, this previously discontinuous and irregular spectrin-actin network becomes smooth and continuous along fiber cell membranes, likely due to stabilization via Tmod1. Unlike other organelles or proteins that are degraded or, indeed, cytoskeletal elements that are proteolyzed/modified, both F-actin and Tmods remain undegraded and associated with mature fiber cell membranes. This suggests that the actin network has a fundamental role in stabilizing and maintaining overall membrane integrity.
Tmod1 and γTM-mediated stabilization of the spectrin-actin network is critical for its long-range connectivity in fiber cells. In this way, the network is essential for maintaining regular hexagonal fiber cell morphology and packing geometry. Loss of Tmod1 leads to F-actin disassembly, reduced levels of γTM, and widespread disruption of the spectrin-actin lattice in differentiating fiber cells, including abnormal membrane protrusions and cell shapes and disordered packing geometry. This demonstrates the importance of the actin cytoskeleton in establishing fiber cell architecture during differentiation. However, transparency remains unaffected in Tmod1-null lenses. Maintenance of the actin cytoskeleton in mature fiber cells may rely on appropriate interactions with lens crystallins, particularly β- and γ-crystallins. γ-crystallins may stabilize F-actin in mature lens fiber cells by protecting against depolymerization. Additionally, optimal levels of HSPB1, a heat shock protein and molecular chaperone that interacts with and stabilizes the actin cytoskeleton, appears to be essential in preventing cataractous changes. The RNA granule component, TDRD7, is necessary to maintain optimal HSPB1 levels, as reduced levels of HSPB1 in Tdrd7 − / − lenses results in abnormal membrane morphology in mature fiber cells and precedes cataract. Moreover, mutations in TDRD7 cause pediatric cataract.
Intermediate filaments: In the lens, intermediate filaments are key regulators of fiber cell architecture and mechanical properties ( Fig. 5.10 ), with vimentin and “beaded filament” proteins, CP49 (Beaded Filament Structural Protein 1 (BFSP1)/phakinin) and filensin (BFSP2), being the most highly expressed. Vimentin filaments are tightly associated with the plasma membrane of lens epithelial and differentiating fiber cells, but are not present in nuclear fiber cells. In contrast, beaded filaments are predominantly associated with fiber cell membranes. This association is, however, dependent on the stage of fiber cell differentiation and can be influenced by proteolytic fragmentation and phosphorylation of the filaments. Accordingly, in the deeper cortex, these proteins become more cytoplasmic. CP49 or filensin gene deletion leads to subtle, age-dependent opacification and loss of optical quality in mice, as detected by slit-lamp examination and laser ray tracing. However, mutations in CP49/BFSP2 protein lead to hereditary cataracts in humans and mice. In contrast, whereas loss of vimentin does not elicit phenotypic changes, vimentin overexpression does induce cataract formation. It is likely that tight regulation of intermediate filaments via post-translational modifications and membrane-cytoplasmic shifts is critical in maintaining the optical properties of the lens.

Intermediate filaments can serve as mechanical support and scaffolding for membrane-associated proteins. Through their plasma membrane associations they are able to maintain the correct spatial organization and plasma membrane profile of fiber cells in the lens ( Fig. 5.10 ). Indeed, the plasma membranes of lens fiber cells are enriched in potential intermediate filament–binding proteins. Changes to the complement of intermediate filament proteins in the lens, seen as a sharp transition from vimentin to beaded filament expression in the lens cortex, appears to be associated with the remodeling of the plasma membrane. Indeed, post-translational modifications and truncations of filensin are observed following its expression in the inner cortex of the bovine lens. In the human lens, this transition occurs rapidly in the remodeling zone (RZ), a narrow area of regulated differentiation in the lens cortex in which fiber cells undergo extreme morphologic changes. The expression of beaded filaments in the RZ may indicate their involvement in membrane deformation/remodeling and/or the establishment of ball-and-socket joints (see further), which first arise in the RZ. Alternatively, alterations in membrane-filament attachments, for example, through AQP0, may also allow undulating membranes to develop. Moreover, deletion of CP49 or filensin does not alter outer cortical radial fiber cell alignment or formation of membrane protrusions, but fiber cells in the inner cortex display striking morphologic abnormalities, such as failing to maintain their paddle-like membrane protrusions, leading to their gross misalignment. This suggests that intermediate filaments are necessary for the maintenance, but not establishment, of interdigitations and long-range stacking of fiber cells. Therefore, beaded filaments appear to become more associated with the membrane to establish interlocking domains, and the switch between intermediate filaments may be an important tool for the remodeling of membranes observed as a function of fiber cell differentiation.
Intermediate filaments help to maintain lens transparency through their association with protein chaperones. Indeed, α-crystallins appear to be critical for the assembly, maintenance, and remodeling of beaded filaments. The dramatic cellular rearrangements that occur in the human RZ are also most likely due to alterations in crystallin associations with beaded filaments. In turn, this association stabilizes the high protein concentrations, which are essential in creating the GRI, thereby maintaining protein homeostasis and transparency. Indeed, recent work has shown that CP49 and filensin proteins are essential components of the super molecular structure in the lens core. Mutations in the CP49 gene or filensin KO prevented the formation of reversible cold cataracts in the lens core at 25°C and reduced the severity of the cataracts at 4°C, suggesting that aggregation or accumulation of these proteins was necessary to precipitate cataractous changes. Interestingly, beaded filaments were not necessary for cold cataracts that extended to the inner fiber cells.
Interactions between cytoskeletal networks: Given their overlap in expression and function, it is perhaps not surprising that the spectrin-actin network and beaded filaments work in functional synergy to regulate three-dimensional hexagonal fiber cell packing, lens transparency, and mechanical stiffness. First, the spectrin-actin network and beaded filament cytoskeleton are biochemically coupled as Tmod1, actin, CP49, and filensin, and they coexist in a protein complex. Second, both networks are also functionally coupled, as deletion of both Tmod1 and CP49 causes defects in fiber cell organization, light scattering, and compressive mechanical properties that are distinct from those defects arising from loss of either Tmod1 or CP49 alone. Both proteins are also needed for the normal formation or stabilization of the small protrusions at the vertices of mature fiber cells. The cytoskeleton also seems to influence the biomechanical properties of the lens in response to application of an external load. The spectrin-actin membrane skeleton appears to mechanically stiffen the lens at low loads and strains, while beaded filaments provide fortification at high loads and strains. Based on these experiments, it has been speculated that the distinct cytoskeletal architectures of the lens epithelium, superficial cortex, deep cortex, and nucleus give rise to radially variable and depth-dependent recruitment of cytoskeletal scaffolding during tissue compression, resulting in radial heterogeneity in the compressive modulus. These networks also appear to synergize in promoting the formation of large gap junction plaques that are crucial for normal lens ion and fluid homeostasis in differentiating fiber cells. Gap junction plaques rest in gaps within the spectrin-actin network along fiber cell membranes, suggesting that the actin skeleton plays a role in the accretion or stability of large gap junction plaques.
Taken together, the observed age-dependent changes in cytoskeletal architecture and the complement of membrane junctions, which alter fiber cell morphology at the ultrastructural and cellular level in different regions of the lens, have the potential to significantly influence the overall optical properties of the lens at the tissue level.
Effects of regional differences in fiber cell morphology on lens transparency, optics, and biomechanics
Light scattering in biological tissue occurs at boundaries between compartments of differing refractive index, such as at the cell border or within the cell, where cellular organelles tend to have different (usually higher) refractive indices. It might be expected therefore that light passing through the lens would be scattered at every interface between cellular and extracellular compartments, and consequently that the lens would be opaque. This is not the case in the outer cortex because differentiating secondary lens fiber cells are initially packed in a geometrically ordered array that helps to minimize light scattering and promote tissue transparency. This level of cellular organization is facilitated by the characteristic flattened hexagonal cross-section profile of fiber cells ( Fig. 5.11A,B ), which serves to not only minimize the extracellular space but also to form a lattice-like structure that acts like a diffraction grating. The regular spacing of fiber cells results in individual light scattering-centers that produce constructive interference in the forward direction and destructive interference at other angles ( Fig. 5.11C ). In this way, extracellular space is minimized and tissue transparency is facilitated, despite the presence of a refractive index mismatch between the cortical fiber cell plasma membrane and the cytosol of cortical fiber cells.

However, the ordered architecture of cells in the outer cortex is not as apparent in the deeper regions of all lenses, with this change in cellular order being most noticeable in humans. In the human lens, the regular cellular order seen in the outer cortex is abruptly disrupted as differentiating fiber cells enter the RZ where fiber cells lose their characteristic hexagonal profile and cell interfaces become unusually interdigitated and irregular. However, cell integrity and gap junctional interactions are not compromised, indicating that this abrupt transition is a highly regulated process. After exiting this lens region, secondary lens fiber cells continue to differentiate, becoming more deeply buried in the lens. Cross-sectional profiles of these mature fiber cells become increasingly circular, the columnar arrangement becomes less apparent, and central fiber cells undergo compaction and exhibit a reduction in the number of fiber cell interdigitations. Hence, in the lens nucleus, fiber cells have little spatial order and exhibit irregular membrane surfaces ( Fig. 5.11D,E ), and the minimization of light scattering is no longer a result of an ordered cellular architecture ( Fig. 5.12F ). Instead, the reduction in light scattering is thought to be the consequence of proteomic and lipidomic changes that occur during lens fiber cell maturation, which produces more closely matched refractive indices between the cytoplasm and plasma membrane of mature fiber cells, thus removing the physical basis for light scattering in the lens nucleus. Overall, it appears that the spatial order of fiber cells is important to establish lens transparency in the outer cortex, whereas it is less important in the deeper regions of the lens.

Establishment of a gradient of refractive index
The GRI contributes to the optical power of the lens and is essential for sharp vision, since it generates a negative spherical aberration to compensate for the positive spherical aberration (see Box 5.1 ) introduced by the cornea and its own geometry. The shape and magnitude of the GRI changes throughout life. In early childhood, the GRI adopts a parabolic shape, but with advancing age a gradual flattening of GRI profile gradient occurs that results in the formation of a central plateau that contributes to an age-related reduction in overall lens power. The GRI is established by varying the ratio of water to protein in the different regions of the lens ( Fig. 5.12A ). This is achieved by a combination of the age-dependent expression of a variety of crystallin proteins that exhibit different refractive increments (dn/dc), the formation of membrane fusions that normalize protein concentrations between growth rings, and the active removal of water from the lens nucleus.
Contribution of the crystallin proteins to the gradient of refractive index
The synthesis and accumulation of very large amounts of crystallin proteins is a major characteristic of lens fiber cell differentiation. As much as 40% of the wet weight of the lens fiber cell can be accounted for by crystallins, a protein concentration that is about three times higher than in the cytoplasm of typical cells. Crystallin proteins can be classified as either “classical” or “taxon-specific.” Taxon-specific crystallins, as the name implies, are found in the lenses of different taxonomic groups. Taxon-specific crystallins can be functional enzymes or proteins that are structurally very similar to enzymes but with little or no enzymatic activity. The classical crystallins that are found in all vertebrate lenses are comprised of two α-crystallins and several members of the beta/gamma (β/γ)-crystallin superfamily. These classical crystallins can be distinguished by their refractive index increment, dn/dc, a variable that defines the contribution of a given concentration of protein to the refractive index of the whole solution, and their intramolecular packing properties, which promotes their solubility in the highly compacted lens nucleus.
α-Crystallin : The human lens expresses two α-crystallin genes, αA and αB. Examination of the protein structure of the α-crystallins revealed that they are members of the widely distributed family of small heat shock proteins. Small heat shock proteins can act as chaperones that stabilize proteins that are partially unfolded and prevent them from aggregating. The ability of α-crystallins to act as a chaperone and prevent protein aggregation and precipitation has been demonstrated in experiments performed in vitro. A similar in vivo role for αA-crystallin has been inferred in mice in which the αA-crystallin gene was disrupted. The function of the α-crystallins in preventing protein aggregation has obvious importance for the lens, because the proteins in lens fiber cells must persist for the life of the individual, and excessive protein aggregation could lead to light scattering and cataract formation.
α-Crystallins are also enzymes because they possess serine-threonine auto-kinase activity. It is not yet clear whether the α-crystallins phosphorylate other proteins in lens fiber cells. The α-crystallins are themselves normally phosphorylated in vivo and can be phosphorylated in vitro; however, the factors that regulate the phosphorylation of α-crystallins and the importance of this phosphorylation for the function of the α-crystallins in the living lens has yet to be determined (see ref ).
α-Crystallin proteins normally associate in the lens cell cytoplasm to make high-molecular-weight complexes containing approximately 30 subunits. The structure of these complexes has been revealed by cryoelectron microscopy. These observations show that native α-crystallin complexes can be assembled in a number of configurations, indicating that there is substantial flexibility in the way the subunits associate. α-Crystallin monomers also readily exchange between high-molecular-weight complexes, further supporting the view that α-crystallin complexes are quite plastic.
The phenotype of αA-crystallin knockout mice provides insight into the function of α-crystallins in vivo. The lenses of these animals are slightly smaller than normal but structurally quite similar to the normal lens. Mature fiber cells contain aggregates of proteins that lead to the formation of cataracts, beginning a few weeks after birth. Analysis of these aggregates shows that they contain large amounts of αB-crystallin and smaller amounts of other proteins. These results suggest that, in the fiber cell cytoplasm, αA-crystallin is partly responsible for preventing αB from aggregating. In addition, when lens epithelial cells from these animals were cultured in vitro, they grew more slowly than normal cells, were more sensitive to stress, and had a higher rate of apoptosis, accounting for the smaller size of the knockout lenses. Therefore, αA-crystallin appears to be important for the normal function of lens epithelial and fiber cells. A naturally occurring mutation in the αB-crystallin gene (CRYAB) leads to the formation of cataracts and “desmin-related” myopathy. In vitro tests showed that the mutant form of the protein had no chaperone activity and even enhanced the aggregation of test proteins. These studies suggest that αB-crystallin has important chaperone functions in the lens and in other cells of the body. However, the fact that the mutant protein accelerated the denaturation of test proteins leaves open the possibility that the mutant is a gain-of-function. In this case, the cataracts and myopathy seen in individuals carrying this mutation could be due to the destabilizing function of the protein, rather than loss of its function as a chaperone. However, additional mutations in CRYAB, which do not have myopathy as part of their phenotype, support the view that loss of its chaperone function contributes to cataract.
β- and -γ crystallins : The β/γ crystallin superfamily is more diverse than the α-crystallins. Originally thought to be two distinct protein families, subsequent protein sequencing of the β- and γ-crystallins shows them to be closely related. The major difference in these proteins is the tendency for most of the β-crystallins to form multimers, whereas the γ-crystallins exist as monomers. Solving the three-dimensional structure of these molecules confirmed their close structural relationship. It also confirmed that the N- and C-terminal extensions of the β-crystallins provided a structural rationale for why these family members form higher-order complexes. There are six β-crystallin polypeptides (βA1, βA3, βA4, βB1, βB2, βB3) and three gamma (γ) crystallins (γS, γC, γD) expressed in the human lens, although the β A1 and βA3 polypeptides are derived from the same gene (CRYBA1). β- and γ-crystallins bind calcium in vitro and may buffer this important cation in the lens fiber cell cytoplasm.
γ-crystallins are thought to function in the lens as structural proteins, and γ-crystallin has the highest dn/dc increment (~0.199 versus ~0.193 for α-crystallin) of the classical crystallin proteins. Because γ-crystallins are differentially expressed in the lens nucleus during prenatal growth, they are the predominant crystallin protein expressed in the nucleus of the human lens. It is likely that this expression pattern combined with their inherently higher dn/dc values ensures that the refractive index is highest in the lens nucleus.
In addition, structural features of γ-crystallins allow intramolecular packing that results in a highly compact form facilitating the short-range spatial order that supports lens transparency. nterestingly, using a model of noninteracting hard spheres, Zhao and colleagues showed that the higher macromolecular dn/dc afforded by the γ-crystallins can significantly reduce the concentration of protein required to achieve the GRI, which then has the effect of reducing osmotic pressure generated by the proteins and their propensity to aggregate. As such, this property of γ-crystallins allows these extremely long-lived proteins to remain soluble in the highly compacted lens nucleus while delivering the required refractive power. γ-crystallins are also known to have unusually low frictional ratios, suggesting that they may have lower tendencies for “sticky” interactions with other proteins, which is relevant in a polydisperse and crowded lenticular environment. This property is likely due to highly conserved tertiary features and the close packing of surface side chains. Therefore, a key role for γ-crystallins may involve acting as a “molecular lubricant” to maintain short-range order by separating distinct components of the lens. This avoids the formation of light-scattering groups through protein unfolding, aggregation, or phase separation. Moreover, the high level of intracellular crowding in the lens leaves little space for protein unfolding and can provide additional stabilizing forces.
In addition, it has recently been suggested that the short-range order of γ-crystallins can be modulated by the presence of other molecules. For instance, ATP can act as a hydrotropic molecule that helps prevent protein aggregation, in addition to its established role as a cellular energy source. Hydrotropes are amphiphilic molecules that at millimolar concentrations solubilize hydrophobic molecules, preventing protein aggregation in aqueous solution. By shielding the hydrophobic regions of protein molecules, ATP provides a hydrophilic interfacial surface comprised of the negatively charged triphosphate side chains of ATP molecule. This hydrophilic surface in turn forms an interfacial dynamic water layer that has been proposed to separate adjacent lens fiber cell proteins, keeping them from aggregating. The hydrotropic function of ATP in preventing protein aggregation would perhaps provide an explanation for the extremely high concentrations of ATP found in the lens, and an age-related decline in ATP concentrations in the center of the lens could contribute to the protein aggregation observed in nuclear cataract in the elderly.
The higher-order structure and dn/dc properties of proteins are the result of their primary amino acid structure. In this regard, lens crystallins have highly unusual amino acid compositions compared with other proteins. β/γ-crystallins contain Greek key motif domains that are enriched with the amino acids lysine and glutamic acid, which exhibit higher dn/dc values than classical Greek motifs containing arginine and aspartic acid residues with lower dn/dc values. These substitutions may allow for more hydrogen bonding and possibly more compact structures. Additionally, mammalian β/γ-crystallins have a higher proportion of interstrand salt bridges compared with other proteins, suggestive of long-range structural stabilization and interactive potential, highly relevant properties for such long-lived proteins.
In addition to the amino acid composition, it is important to consider the effect that other factors such as protein hydration can have on the high refractivity of lens proteins. The layer of water around a protein, known as its hydration shell, is dynamic and heterogenous. Protein surface solvation provides a large energetic deterrent for protein-protein contacts that may lead to protein aggregation, thereby contributing to protein solubility. Indeed, some cataract-forming mutants of human γ-crystallin are due to changes in local solvation properties rather than protein structure. The behavior of the hydration shell, as it forms hydrogen bonds with multiple exposed amino acid residues, is distinct from that of a solution of its component amino acids and can also be reasonably expected to contribute to the refractive index. Current hypotheses propose that the arrangement of exposed hydroxyl groups on the protein surface may affect protein hydration and, thus, the dipole moment and polarizability. In addition, or alternatively, short-range interactions between highly polarizable amino acids with pi-bonding systems, a feature common in lens crystallins, may cause local regions of anisotropic polarizability. Indeed, accounting for cation-pi and pi-pi interactions improves the agreement between predicted and measured refractive indices.
Role of membrane fusion in the establishment of the gradient of refractive index
The lens consists of a series of discrete growth rings that contain fiber cells, which at specific stages of cell differentiation become metabolically linked by membrane fusions. It has been proposed that these intracellular linkages allow for the exchange of large proteins such as the crystallins within, but not between, adjacent growth rings. This intercellular diffusion of crystallins via membrane fusions serves to equalize the protein concentration, and therefore the refractive index, between cells within a growth ring. However, the lack of fusions between adjacent growth rings ensures that differences in refractive index are maintained between adjacent rings ( Fig. 5.12B ). The presence of discrete concentric growth rings, plus the differential expression of α-, β- and γ-crystallin subtypes with different refractive indices, forms the structural basis for the establishment of the GRI. Although not connected by membrane fusions, adjacent growth rings are coupled by gap junctions channels. Gap junction channels, although not permeable to crystallin proteins, allow the flow of ions and water between adjacent growth rings. It follows, therefore, that the removal of water from the lens nucleus would not only steepen the GRI by increasing the concentration of crystallin proteins but also smoothen the GRI profile established by stepwise regional differences in crystallin subtype expression between the individual concentric growth rings (Fig. 12B). This active maintenance of the GRI and its effect on the refractive properties of the lens are discussed in the next section.
Biomechanical properties of the lens
The mammalian lens appears to exhibit a radial stiffness gradient, with cortical fiber cells gradually stiffening as they age and becoming displaced toward the lens nucleus. The spectrin-actin network is integral for developing and maintaining this mechanical stiffness. Although mechanical loading appears to be distributed throughout the lens capsule, epithelium, and fiber cells, specific microstructures have different propensities to bear and recover from load. For example, despite bearing high levels of axial strain, mouse lens epithelial cell area, capsule thickness, and fiber cell widths recover when the load is removed. The load-bearing properties of epithelial cells may be conferred by the hexagonal actomyosin array, which generates tensional forces. General actomyosin disruption can affect the whole (chick) lens biomechanical response to bear load. In contrast, the separation of fiber cell tips is irreversible at high loads, leading to incomplete recovery of the suture gap area and incomplete whole-lens resilience. Tropomyosins (Tpms) such as Tpm3.5, directly by associating with F-actin and indirectly by promoting Tmod1 and α-actinin association, stabilize the actin network associated with fiber cell membranes. Decreased levels of Tpm3.5 and the accompanying dissociation of Tmod1 led to rearrangement of F-actin networks that decreased lens resilience, i.e., the ability of the lens to resume its original shape after removal of a compressive mechanical load. Curiously, fiber cell organization and morphology remained unaffected. Alternatively, loss of Tmod1 resulted in loss of large fiber cell undulations, or paddles, in mature fiber cells, which was correlated with a decrease in lens stiffness, even at low mechanical loads. In contrast, very old mouse lenses, despite misalignment/loss of characteristic hexagonal profile of outer cortical fiber cells, continue to exhibit an age-dependent increase in stiffness, suggesting that fiber cells packing in this region may not contribute to the biomechanical properties of the lens.
Intermediate filaments are also thought to influence the biomechanical properties of the lens. When subjected to ramp compression and decompression cycles, young CP49-null lenses showed decreased stiffness and slightly increased resilience compared with wild-type lenses. Loss of one or both alleles of aquaporin-0, a beaded filament–binding partner in the lens, led to a significant reduction in the load-bearing ability of the lens, suggesting that aquaporin-0 is required to provide sufficient anchorage for beaded filaments to establish normal lens biomechanics. Interestingly, loss of aquaporin-5 did not affect the compressive load-bearing capacity of the mouse lens. In contrast, loss of Cx46, which forms gap junctions in both the lens cortex and nucleus, resulted in increased stiffness of the mouse lens at young and old ages. This increase in stiffness was alleviated in young, but not old, lenses that lacked CP49. Overall, the appropriate and combined interaction of beaded filaments with plasma membrane–associated proteins and junctional complexes appears to be important in maintaining the biomechanical properties of the lens as it ages, although the exact mechanisms linking these molecular changes to gross morphology remains unknown.
Metabolic determinants of the transparent and refractive properties of the lens
While the structural organization of the lens establishes its transparent and refractive properties, the lens is not a passive optical element, but a biologic tissue that requires the input of energy to drive the structural and function processes that actively maintain its optical properties. Because of its position in the eye beyond the eye-blood barrier ( Fig. 5.1 ) and its internal structural organization into morphologically distinct regions ( Fig. 5.3 ), the different regions of the lens do not have equal access to the nutrients supplied to it via the aqueous and vitreous humors and thus exhibit different metabolic requirements. Fiber cells in the outer cortex are closer to the nutrient supply and contain functional mitochondria, which allows them to use aerobic metabolism to meet the higher energy demands required to perform the multitude of cellular processes associated with the massive elongation of these differentiating cells. In contrast, deeper mature fiber cells that are internalized within the lens are further from the nutrients in the humors that bathe the surface of the lens and, having lost their mitochondria, rely on anaerobic glycolytic metabolism to produce the concentrations of ATP and other reducing equivalents for the antioxidant enzyme systems that operate to preserve the solubility of the crystallin proteins. How nutrients are delivered to different regions of the lens from the surrounding humors is considered later in the chapter, but first the major metabolic functions of the different lens regions are outlined.
Glucose metabolism
The primary source of energy for the lens is glucose, which in the absence of a blood supply is supplied to the lens directly from the surrounding aqueous and vitreous humors, which in humans have glucose concentrations of approximately 3.2 mM and 3.0 mM, respectively. Although the normal concentration of glucose within the lens varies between species, it is in the order of 10 mg/100 g tissue. Like in other tissues, glucose in the lens is primarily metabolized by three pathways: glycolysis, the pentose phosphate pathway (hexose monophosphate shunt), and the polyol pathway. However, it appears that the relative activity of these pathways may vary in the different lens regions. As the initial step in a number of metabolic pathways, glycolysis is essential for the maintenance of lens physiologic function and tissue transparency over many decades of life, since perturbations to major enzyme-mediated steps in glycolysis result in lens swelling and cataract. Owing to the differentiation-dependent degradation of cellular organelles, aerobic glycolysis and metabolism via the citric acid cycle is possible only in the lens epithelium and peripheral fiber cells that still contain mitochondria.
Hence, aerobic metabolism only accounts for approximately 20% to 30% of the total lens ATP production while consuming only around 3% of the glucose supplied to the lens. The remaining 70% of lens glucose metabolism is carried out under anaerobic conditions, which produces lactate that is thought to contribute to a measurable pH gradient from the periphery to the center of the lens. In addition, owing to the lack of a blood supply, the oxygen concentration within and around the lens is much lower than in most other parts of the body. This, in combination with the consumption of oxygen by mitochondria in epithelial and differentiating fiber cells, means that an oxygen gradient is established across the lens with the negligible oxygen levels being measured in the lens nucleus.
In the lens, the pentose phosphate pathway uses glucose to produce a number of reducing equivalents (NADPH) that help protect against oxidative stress by reducing oxidized glutathione (GSSG) to regenerate glutathione (GSH). NAPDH is also used in the human lens by the polyol (sorbitol) pathway. In this pathway, sorbitol is formed from glucose by aldose reductase (AR) using NADPH as a cofactor, which is then converted to fructose by a second enzyme, polyol (sorbitol) dehydrogenase, using nicotinamide adenine dinucleotide (NAD + ) as a coenzyme. Under normal physiologic conditions, almost one-third of the glucose entering the human lens is metabolized through this sorbitol pathway. In the human lens, the majority of AR, and its activity, is present predominantly in the epithelium. In diabetes, the elevated levels of glucose in the blood, and hence the aqueous humor, have been linked to hyperglycemia-related changes to the lens and cortical cataract formation. Sorbitol is osmotically active, and therefore an overabundance of sorbitol can draw additional water inside fiber cells, which, by causing cellular swelling and damage, disrupts the ordered tissue architecture of the lens and results in light scattering. Whereas this mechanism has been demonstrated in species such as dogs and rats, AR activity in the human lens is lower. Hence, the exact mechanism of diabetic cataract in humans is less clear and is thought to involve an oxidative component in addition to osmotic stress.
Antioxidant defense systems
Molecular oxygen is, directly or indirectly, the source of most oxidative damage. If cells could survive in an atmosphere free of oxygen, most oxidative damage would be avoided. For most cells this is not possible, but the oxygen tension around the lens in the living eye is quite low, less than 15 mmHg (~2% O 2 ) just anterior to the lens and less than 9 mmHg (~1.3% O 2 ) near its posterior surface. Oxygen levels within the human lens are even lower (<2 mmHg). The low oxygen tension around and within the lens helps to protect lens proteins and lipids from oxidative damage. However, even with this low level of oxygen, the lens normally derives a proportion of its ATP from oxidative phosphorylation, a process that inherently generates free oxygen radicals.
Hydrogen peroxide is another molecule that has been suggested to cause oxidative stress to the lens. Hydrogen peroxide is produced in mitochondria by the enzyme superoxide dismutase acting on superoxide anions, a by-product of oxidative phosphorylation. Hydrogen peroxide can also be produced during the oxidation of ascorbic acid, which is present at high levels in the aqueous and vitreous humors (~1.5–2.5 mM) that bathe the lens. Both processes may contribute to the resting hydrogen peroxide levels that have been reported within both intraocular fluids and the lens itself. The level of hydrogen peroxide in the aqueous humor has been reported to average over 30 mM and to exceed 200 mM in about one-third of cataract patients. The lens has two enzyme systems to detoxify hydrogen peroxide. Lens epithelial cells have abundant levels of catalase, which converts hydrogen peroxide to water, and GSH peroxidase, an enzyme that couples the reduction of hydrogen peroxide to the oxidation of GSH. Studies on cultured lenses and lens epithelial cells suggest that GSH peroxidase provides most of the protection against the potential damaging effects of physiologic levels of hydrogen peroxide, as catalase is only effective against relatively high concentrations of peroxide.
The lens has a number of antioxidants, but the principal antioxidant in the lens is GSH. It has been shown that the maintenance of GSH in the lens nucleus is critical to the prevention of protein crosslinking, crystallin aggregation, and light scattering. GSH is a tripeptide of the amino acids glutamine, cysteine, and glycine, and provides most of the protection against oxidative damage in the lens. It can prevent the oxidation of components of the lens cytoplasm because its concentration in the lens is very high, approximately 4 to 6 mM, and its sulfhydryl group is readily oxidized. These high intracellular GSH levels are maintained by a combination of pathways ( Fig. 5.13 ), which includes (1) synthesis of GSH from its precursor amino acids, cysteine, glutamate, and glycine, by the sequential actions of the enzymes glutamate cysteine ligase (GCL) and GSH synthetase; (2) transport of GSH from outside the cell via specific carriers; (3) regeneration of GSH from oxidized GSH (GSSG) by the enzyme GSH reductase (GR) and nicotinamide adenine dinucleotide phosphate (NADPH) generated via the pentose phosphate pathway; and (4) the export of GSH, followed by its degradation into constituent amino acids by γ-glutamyl transpeptidase (GGT) to ensure GSH turnover. In the absence of GSH synthesis in the lens nucleus, GSH needs to be regenerated from GSSG. GSH can form disulfide bonds with the oxidized sulfhydryl groups of proteins. These GSH-protein mixed disulfides can then be reduced by a second molecule of GSH, a process that is facilitated by the enzyme thioltransferase. This regenerates the protein sulfhydryl and forms GSSG, which can then be subsequently reduced to regenerate GSH. When GSH levels have been lowered in lens epithelial cells or whole lenses, cell damage and cataract formation follow rapidly. Furthermore, it has been shown in human and rat lenses that GSH-dependent enzymes protect and restore protein-thiol groups in their reduced state to prevent protein crosslinking.

In addition to GSH, ascorbic acid is used to protect the lens against oxidative damage. Ascorbate is actively transported from the blood to the aqueous humor by a sodium-dependent transporter located in the ciliary epithelium and reaches concentrations in the aqueous humor that are 40 to 50 times higher than levels in the blood. The ascorbate levels in the lens and other intraocular tissues are also substantial. Dehydroascorbate (DHA), the oxidized form of ascorbic acid, enters lens cells by way of the glucose transporter (GLUT), where it is reduced by GSH-dependent processes. Like GSH, ascorbate is readily oxidized, forming DHA in the process. Therefore ascorbate can react with free radicals and other oxidants in the aqueous humor and the lens, preventing these molecules from damaging lens lipids, proteins, and nucleic acids. However, if DHA accumulates in the lens, its metabolites can react with lens proteins, increasing lens color and decreasing protein stability. The high GSH levels in the lens likely maintain the majority of ascorbate in its reduced state, thereby avoiding much of this potential damage.
Ultraviolet protection
Lens antioxidant defense systems are also important to support the human lens’ role as an ultraviolet (UV) filter that works in conjunction with the cornea to protect the retina against light-induced damage from wavelengths of light in the UV range (250–400 nm). While the cornea restricts the transmission of wavelengths less than 290 nm, the remaining harmful solar radiation is absorbed by UV filters in the lens that absorb light in the 295 to 400 nm range. These filters are small molecules formed through tryptophan metabolism and, although present in many mammals, are predominantly found in primates and humans.
The major UV filter compounds found in the human lens are kynurenine (Kyn), 3-hydroxykynurenine (3-OHK), and 3-hydroxykynurenine O-β-D-glucoside (3-OHKG). They are found at higher concentrations in the nucleus than the cortex, and their concentrations decline with advancing lens age. These compounds can undergo spontaneous deamination to produce the structurally related UV filters, which follow the same age-related concentration changes observed for the kynurenine UV filters. Some of these UV filters are unstable at physiologic pH and undergo spontaneous degradation or react with a range of other small molecules found in the lens. For example, 3-OHKG can undergo deamination to produce deamidated 3-hydroxykynurenine O-β-D-glucoside (3OHKG-D) and 3-hydroxykynurenine O-β-D-glucoside yellow (3OHKG-Y). For example, GSH reacts with 3-OHKG to form glutathionyl-3-hydroxykynurenine glucoside (GSH-3-OHKG), a UV filter and lens fluorophore that increases in concentration in the lens nucleus with increasing lens age. In addition, UV filters are present in cataractous tissue at concentrations higher than age-matched controls, and in modified forms. For this reason, they have been implicated in cataract formation, possibly through their modification of crystallin proteins, and subsequent action as photosensitizers that increase oxidative stress in the aging lens.
Physiological determinants of the transparent and refractive properties of the lens
The structural and metabolic adaptations described previously that establish the transparent and refractive properties of the lens in turn impose a number physiologic constraints on the lens. As a consequence of these constraints, the lens requires a specialized transport system that can: (1) rapidly deliver nutrients/antioxidants to, and remove metabolic wastes from, deeper-lying fiber cells, so that the solubility of lens crystallins is maintained and protein aggregation prevented; (2) impose the negative membrane potential that is required to maintain the steady-state volume of the fiber cells, which is necessary to prevent changes in their cellular morphology and light scatter; and (3) prevent alterations to the GRI by countering water influx into the lens generated by the high concentrations of the lens crystallins needed for the lens to refract light while immersed in aqueous media. Our understanding of how the cellular physiology of the lens regulates these key features has evolved over recent decades as advances in histologic and electrophysiological techniques, utilized to study fluid transport in other epithelial tissues, have been applied to the lens. A major early milestone came from the work of Robinson and Patterson who used vibrating probe measurements to show that a standing flow of ionic current, which was directed inward at the poles and outward at the equator, was a common feature of different species of vertebrate lenses. Utilizing a combination of electrical impedance measurements and theoretical modeling, Mathias subsequently proposed that these currents measured at the lens surface represent the external portion of a circulating ionic current that drives a unique internal microcirculatory system, which operates to maintain fiber cell homeostasis and therefore lens transparency. Although the microcirculation model of lens transport was not initially accepted by all, in recent years the application of other experimental techniques to the lens has allowed researchers to actually measure and visualize the solute and fluid fluxes original predicted by the microcirculation system model. These findings have significantly strengthened and expanded our current understanding of how the underlying cellular physiology of the lens actively maintains its transparency and refractive properties.
An overview of the lens microcirculation system
Mathias et al. originally proposed a working model in which circulating Na + currents, initially identified by vibrating probe measurements, enter the lens at both the anterior and posterior poles via an extracellular pathway located between fiber cells ( Fig. 5.14A ). Na + eventually crosses the fiber cell membranes, and then flows from cell to cell toward the surface via an intracellular pathway mediated by gap junction channels. Because the gap junction coupling conductance in the outer shell of differentiating fibers is concentrated at the equatorial plane of the lens, this intracellular current is directed toward the equatorial surface of the lens where epithelial and peripheral fiber cells that contain the highest densities of Na + /K + pumps are located, to actively transport Na + out of the lens. The driving force for these fluxes is hypothesized to be the difference in the electromotive potential of surface cells and inner fiber cells ( Fig. 5.14B , top panel). Data from ion substitution experiments performed on both intact lenses and isolated fiber cells have shown that the surface cells, including epithelial cells and newly differentiating fiber cells, contain Na + /K + pumps, and K + -channels, which together generate a negative electromotive potential. Fiber cells deeper in the lens lack functional Na + /K + pumps, and K + -channels and their permeability is dominated by nonselective cation and Cl − conductances. In these inner cells, the negative membrane potential that helps maintain the steady-state volume of the fiber cells is imposed on deeper fiber cells by virtue of their connection to surface cells via gap junctions. This electrical connection, together with the different membrane properties of the surface and inner cells, causes the standing current to flow.
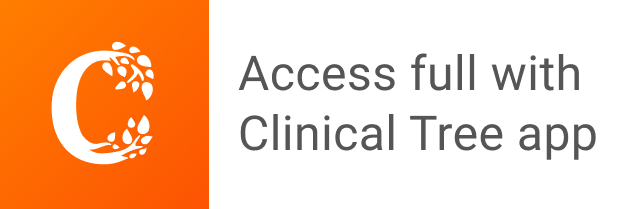