The lens is a remarkably specialized epithelial tissue that is responsible for fine-tuning the image that is projected on the retina. To perform this function it must be transparent, have a higher refractive index than the medium in which it is suspended, and have refractive surfaces with the proper curvature. Because the refractive power of the lens is variable, it permits the diopteric apparatus to focus on objects that are near or far.
To maintain transparency and a high refractive index, lens fiber cells are precisely aligned with their neighbors, have minimal intercellular space, and accumulate high concentrations of cytoplasmic proteins, the crystallins. Disruption of the precise organization of the lens fiber cells or aggregation of the proteins within them can destroy the transparency of the lens – a process known as cataract formation. Cataracts are the leading cause of blindness worldwide and the removal of cataracts is the most common surgical procedure in the aged population.
This chapter provides an overview of the structure, development, biochemistry, physiology, and pathology of the lens. Information about the human lens is emphasized, although studies using animals are included when data are not available for humans. Information is provided on the causes of cataracts and the mechanisms that are thought to protect the lens from cataract. The unsolved issues in the biology and pathology of the lens are highlighted throughout. References to comprehensive reviews and original source material are included to assist the reader. The citations provided are not meant to be inclusive, but to highlight articles of special relevance.
The anatomy of the adult lens
The lens is formed from two populations of specialized epithelial cells ( Fig. 5.1 ). A sheet of cuboidal cells, the lens epithelium, covers the surface of the lens closest to the cornea. The bulk of the lens consists of concentric layers of elongated fiber cells. The outer shells of fiber cells extend from just beneath the epithelium to the posterior lens surface, a distance of over 1cm in adults. An elastic extracellular matrix, the lens capsule, which is secreted by the epithelial and superficial fiber cells, surrounds the entire lens. In the adult lens, most epithelial cells and all fiber cells are not dividing. Cells near the equatorial margin of the lens epithelium, in a region called the germinative zone, proliferate slowly. Most of the cells produced by mitosis in this region migrate towards the posterior of the lens and differentiate into fiber cells at the lens equator. These new fiber cells elongate and accumulate large amounts of crystallins. During elongation the posterior (basal) ends of the fiber cells move along the inner surface of the capsule and their anterior (apical) ends slide beneath the epithelium until they meet elongating cells from the other side of the lens near the posterior and anterior midlines ( Fig. 5.1 ). The junctions between the ends of cells from the opposite sides of the lens are called the sutures. Once fiber cells reach the sutures they stop elongating and their basal ends detach from the capsule ( Fig. 5.1 , inset). Soon after reaching the sutures, fiber cells degrade all intracellular membrane-bound organelles, including their nuclei, mitochondria, and endoplasmic reticulum. Mature fiber cells are gradually buried deeper in the lens as successive generations of fibers elongate and differentiate. In this way the lens continues to increase in size and cell number throughout life. Because protein synthesis ceases just before organelle degradation, the components of mature fiber cells must be much more stable than those in cells found in other parts of the body. In fact, since the fiber cells in the center of the lens are present at birth and persist until death (or cataract surgery), their constituent proteins and membranes may last for more than 100 years.

The lens is suspended in the anterior of the eye by a band of inelastic microfibrils, the zonules, which insert into the lens capsule near the equator ( Figs 5.1 & 5.2 ). These fibrils originate in the non-pigmented layer of the ciliary epithelium, a tissue that is located just posterior to the iris. They are composed of the protein fibrillin, one of the components of elastic fibrils in many connective tissues throughout the body. , However, the zonules do not stretch appreciably. Changes in the tension applied to the zonules are responsible for the alterations in lens curvature that occurs during accommodation, the process by which the lens focuses on objects nearby or at a distance.

The basics of lens refraction and transparency
The refractive properties of the lens are the result of the high concentration and graded distribution of crystallin proteins in the cytoplasm of the lens fiber cells and the curvature of the lens refractive surfaces. Lens crystallins accumulate to concentrations that are up to three times higher than in typical cells. This gives the fiber cells a significantly higher refractive index than the fluids around the lens. In the emmetropic eye the curvature of the refractive surfaces of the lens focuses light at the photoreceptors of the retina. The curvatures of the lens surfaces are the result of the tension on the zonules, the elasticity of the capsule, and the growth properties of the lens fibers and epithelial cells. In younger individuals refractive error, myopia or hyperopia is often caused by abnormalities in corneal curvature or the length of the globe, but rarely by defects in the curvature or refractive index of the lens.
Transparency depends on minimizing light scattering and absorption. Light passes smoothly through the lens as a result of the regular structure of lens fibers ( Fig. 5.3 ), the absence of membrane-bound organelles in the deeper fiber cells, and the small and uniform extracellular space between the fiber cells. Paradoxically, the high crystallin concentration of the lens fiber cell cytoplasm is an essential component of lens transparency. Reduced light scattering is due to short-range interactions between the highly concentrated crystallins. , Although it absorbs increasing amounts of the shorter wavelengths of visible light as it ages, giving the lens a pale yellow to brown color, the young human lens is nearly colorless. ,

The early development of the lens
The cells that form the lens are originally part of the surface ectoderm covering the head of the embryo. Interactions between the future lens cells and nearby tissues during early development give these cells a “lens forming bias.” As a result of these interactions, patches of cells that lie on either side of the head are marked by expression of the transcription factor, Pax6. At the same time, neural epithelial cells on either side of the diencephalon in the embryonic forebrain bulge laterally to form the optic vesicles, which eventually contact the surface ectoderm cells ( Fig. 5.4A ). The cells of the optic vesicle also express Pax6 and Pax6 function in these cells is essential for eye formation. , Some of the genes that regulate and are regulated by Pax6 at early stages of eye formation are now known, although the number of genes involved and knowledge about their interactions is likely to increase.

Members of the bone morphogenetic protein (BMP) family of secreted factors play an essential role in lens formation. Deletion of the genes encoding BMP4 or BMP7 in the mouse embryo prevents lens formation in most cases (a minority of BMP7 null animals make a small, abnormal lens). Deletion of BMP4 leads to decreased expression of the transcription factor Sox2 in the lens placode, while loss of BMP7 is associated with absence of Pax6 expression. BMP4 appears to be derived primarily by the optic vesicle; implantation of a bead containing BMP4 into the lumen of the vesicle can rescue lens formation in BMP4 knockout embryos. BMP7 mRNA mainly localizes in the pre-lens surface ectoderm, suggesting that BMP7 acts as an autocrine or paracrine factor to promote lens formation.
After they make contact, the optic vesicles and the prospective lens ectoderm cells secrete an extracellular matrix that causes these cell layers to adhere tightly to each other ( Fig. 5.4B ). The surface epithelial cells then elongate, forming the thickened lens placode ( Fig. 5.4B ). Soon afterward, the lens placode and the adjacent cells of the optic vesicle buckle inward to form the lens pit ( Fig. 5.4C ). This morphological transformation is accompanied by the formation of the bi-layered optic cup. The invaginated lens placode soon separates from the surface ectoderm by a process involving the death of the cells in the connecting stalk between the cells of the surface ectoderm and the lens ( Fig. 5.4D ). The Pax6-expressing ectodermal cells adjacent to the lens placode that remain on the surface of the eye become corneal and conjunctival epithelial cells.
During lens invagination, the extracellular matrix between the optic vesicle and the lens begins to diminish and the two tissues separate ( Fig. 5.4E ). , The space that is formed between them is rapidly filled with a loose extracellular matrix, the primary vitreous body, that is secreted by the cells of inner layer of the optic cup ( Fig. 5.4E ).
The epithelial cells that give rise to the lens vesicle originally lie on a thin basal lamina. In the process of invagination, this basal lamina comes to surround the lens vesicle. It gradually thickens by the deposition of successive layers of basal lamina material to form the lens capsule.
Soon after the lens vesicle separates from the surface ectoderm, the cells in the portion of the vesicle that are closest to the retina begin to elongate. The elongation of these primary fiber cells soon obliterates the lumen of the vesicle as their apical ends contact the apical ends of the anterior epithelial cells ( Fig. 5.4E,F ). Primary fiber cell formation establishes the fundamental structure of the lens, with epithelial cells covering the anterior surface and elongated fiber cells filling the bulk of the lens.
At early stages of lens formation, most of the lens epithelial cells are actively proliferating. Cells at the margin of the epithelium are pushed or migrate towards the equator and are stimulated to differentiate into secondary fiber cells by factors present in the vitreous body. As the secondary fibers elongate and their basal and apical ends curve towards the center of the lens, they displace the central primary fibers from their attachments with the capsule and the lens epithelium. This process buries the primary fiber cells in the center of the lens ( Fig. 5.1 ). In the adult lens these cells comprise the “embryonic nucleus.” Deposition of successive layers of secondary fiber cells continues throughout life. The rate of fiber cell formation, and therefore lens growth, is rapid in the embryo and slows greatly after birth ( Fig. 5.5 ).

In addition to Pax6, at least one additional transcription factor may be essential for lens formation. In chicken embryos, prospective lens cells express L-maf, a member of the maf family of b-ZIP transcription factors, soon after they contact the optic vesicle. When modified forms of this protein that bind to DNA but do not activate transcription are expressed in the prospective lens-forming region of chicken embryos, the formation of the lens placode and lens vesicle is suppressed. Expression of L-maf in regions of the head ectoderm outside of the lens placode (but within the area expressing Pax6) leads to the formation of extra lenses. Although members of the maf family of transcription factors are expressed during lens formation in mammals, there is no factor comparable in function to chicken L-maf. Since deletion of individual members of the maf family does not prevent lens formation in mice, multiple maf family members may assume the function of L-maf in mammals.
The proper separation of lens vesicle from the overlying presumptive corneal epithelium is defective in the mouse mutant, dysgenetic lens ( dyl ). A mutation was identified in the gene encoding the “forkhead” transcription factor, Foxe3, in the dyl strain. Foxe3 is selectively expressed in the lens placode and developing lens epithelium. , Lenses lacking Foxe3 show severe defects in lens epithelial cell proliferation and subsequently degenerate. A similar phenotype is seen in lenses lacking the transcription factor, AP-2α. These also fail to separate from the surface ectoderm and show defects in epithelial cell differentiation.
Targeted disruption of the genes encoding three additional transcription factors that are normally expressed during primary fiber cell differentiation, c-Maf, Sox1, and Prox1, causes failure of elongation of primary lens fiber cells. In mice lacking any one of these genes, lens fiber cells do not elongate or express the high level of lens crystallins that are characteristic of primary fiber cells. The diffusible factors that trigger fiber cell formation and the mechanisms responsible for fiber cell elongation and the regulation of crystallin synthesis are discussed below (see Box 5.1 ).
Several genes have been identified that are required for the formation of the normal lens. The proteins encoded by these genes, including Pax6, Pitx3, c-Maf and Foxe3, are transcription factors; they control the expression of batteries of genes required to make a lens. Most of these mutations are dominant and result from “haploinsufficiency;” loss of the function of one copy has a severe effect on lens development. Defects may range from mild cataracts to absence of the lens. Many of these mutations are associated with defects in the development of the anterior segment, including the corneal endothelium and stroma and the aqueous humor outflow pathway. Anterior segment defects can be secondary to Peters anomaly, the failure of the lens to separate from the surface ectoderm, or to less obvious interactions between the developing lens and the mesenchymal cells that form the structures of the outflow pathway.
Mice homozygous for the aphakia mutation form lens vesicles, but the lens cells degenerate soon after. The aphakia mutation was mapped close to the gene encoding a homeodomain transcription factor, Pitx3, that is expressed early in lens development. , Two families were described in which affected individuals have hereditary congenital cataracts and point mutations in Pitx3. Deletions were found in the region upstream and in the coding sequence of Pitx3 in the aphakia strain. , A microarray study indicated that Pitx3 mRNA expression is decreased in lenses lacking a single copy of Pax6.
Lens fiber cell differentiation
Lens fiber cell differentiation is characterized by an exceptional degree of specialization. During their differentiation, fiber cells withdraw from the cell cycle, elongate greatly, express large amounts of proteins (the crystallins), acquire several specializations of their plasma membranes, and eventually degrade all membrane-bound organelles.
In mature lenses, the first evidence of fiber cell differentiation is the withdrawal from the cell cycle of epithelial cells near the lens equator. After their last mitosis, these cells move in a posterior direction into the transition zone ( Fig. 5.6 ). This movement has been termed “migration,” but it may simply result from displacement caused by the proliferation of cells in the germinative zone, a kind of “mitotic pressure.” Cells in the transition zone are postmitotic, but have not yet begun to elongate. As they move posteriorly they are exposed to factors that stimulate their elongation. Studies in chicken embryos and newborn mice, in which the lens was rotated so that the epithelial cell faced the vitreous body, first demonstrated the existence of these factors. , Epithelial cells exposed to vitreous humor in this manner elongated like primary fiber cells. Furthermore, chicken embryo lens epithelial cells stopped progressing through the cell cycle within 9 hours after lens rotation, indicating that suppression of lens cell proliferation at the initiation of fiber cell differentiation is dependent on factors from outside the lens.

Proteins that are known to be involved in regulating the cell cycle in many cell types appear to play an essential role in the cessation of lens fiber cell proliferation. Included in this group are the cyclin-dependent kinase inhibitor protein, p57 (KIP2) and the retinoblastoma protein (pRb). Progression through the cell cycle requires the phosphorylation of regulatory proteins, called cyclins, by cyclin-dependent kinases. The cyclin-dependent kinase inhibitor protein, p57, an inhibitor cell cycle progression, is expressed in the early stage of lens fiber cell differentiation, where it is found bound to cyclins. , In lenses that lack the gene for pRb, a well-known regulator of the cell cycle, p57 levels are low and lens fiber cells do not withdraw from the cell cycle. , This finding suggests that pRb is required for the expression of p57 and that at least one function of p57 is to maintain lens fiber cells in the non-proliferating state by complexing with cyclins. Absence of the two main cyclin-dependent kinase inhibitors expressed in the lens, p27 and p57, leads to extensive lens fiber cell proliferation, even in the presence of pRb, confirming that these molecules play an essential role in the withdrawal of fiber cells from the cell cycle. Lenses lacking both p27 and p57 also do not make detectable levels of crystallins, suggesting that the function of these molecules is essential for normal gene expression during fiber cell differentiation. In addition to the requirement for pRb, the transcription factor Prox1 is also required for high levels of p57 accumulation in the nuclei of lens fiber cells. Prox1 is present in lens epithelial cells, but localizes to the cell nucleus at the onset of fiber cell differentiation.
Some of the basic mechanisms responsible for the extensive cell elongation that characterizes lens fiber cell differentiation have been described, but this process is still not well understood. Early studies suggested that the microtubules of the lens fiber cytoskeleton are required for fiber cell elongation. , However, later experiments showed that fiber-like cells could elongate in the absence of microtubules. Whether other components of the cytoskeleton play an essential role in fiber cell elongation in vivo has not been demonstrated.
In chicken embryo lenses, the early stages of lens fiber cell elongation may be driven by an increase in cell volume. In these cells, initiation of fiber cell elongation is accompanied by changes in the ionic permeability of the plasma membrane. The resulting accumulation of potassium and chloride ions in the cytoplasm leads to an osmotically driven increase in cell volume. Continued cell elongation depends on sustained protein synthesis. , In mouse lenses, secondary fiber cells appear to initiate elongation without increasing in volume. The mechanism by which fiber cell elongation is regulated at later stages of their differentiation, especially when fiber cells reach the sutures and stop elongating, has not been studied.
Several growth factors are capable of initiating lens fiber cell differentiation when added to cultured lens epithelia or, in some cases, when over expressed in the lens in vivo. Among these are members of the fibroblast growth factor (FGF) and insulin-like growth factor (IGF) families. Studies in chicken embryos identified IGF-1 as a potent activator of fiber cell differentiation. However, experiments in rats and mice showed that, although IGFs may play some role in fiber cell differentiation, members of the FGF family appear to be more important in mammals and also contribute to fiber cell differentiation in chicken embryos. Studies using mouse lenses deficient in three of the four FGF receptors have conclusively demonstrated that FGF signaling is required for the formation of lens fiber cells. Other soluble factors, especially members of the BMP family, appear to modulate or potentiate the effects of FGFs on lens fiber cell differentiation.
Lens crystallins
The synthesis and accumulation of very large amounts of crystallin proteins is a major characteristic of lens fiber cell differentiation. As much as 40 percent of the wet weight of the lens fiber cell can be accounted for by crystallins, a protein concentration that is about three times higher than in the cytoplasm of typical cells. Crystallin proteins can be classified as either “classical” or “taxon-specific”. The classical crystallins are comprised of two α-crystallins and several members of the beta/gamma (β/γ)-crystallin superfamily. All vertebrate lenses accumulate large amounts of classical crystallins in their fiber cells. In many species, fiber cells also produce large amounts of taxon-specific crystallins (see reviews , ). As the name implies, different taxon-specific crystallins are found in the lenses of different taxonomic groups. Taxon-specific crystallins are functional enzymes or proteins that are structurally very similar to enzymes, but with little or no enzymatic activity. The levels of taxon-specific crystallins sometimes exceed those of the classical crystallins. Adult human lenses do not produce taxon-specific crystallins, although the enzyme betaine-homocysteine methyltransferase is present at high levels in the embryonic nucleus of the rhesus macaque lens, suggesting that this enzyme may serve as a taxon-specific crystallin during the early development of the primate lens (see Box 5.2 ) .
Lens fiber cells accumulate high concentrations of lens-preferred crystallin proteins. Their plasma membranes also have large amounts of protein that form lens-specific gap junctions, water channels or cell–cell adhesions. Mutations in the genes encoding these abundant proteins are responsible for many of the hereditary congenital cataracts that have been identified over the past decade. Most mutations that cause hereditary congenital or juvenile cataracts show a dominant mode of inheritance. Experimental studies in animal models and study of the mutant proteins in cultured cells suggest that the defective proteins encoded by these genes cause cataracts by interfering with the normal function of lens fiber cells or by promoting their own aggregation and, perhaps, the aggregation of normal lens proteins. Therefore, these cataracts are not caused by loss of the normal function of the mutant proteins, but by the acquisition of an abnormal function. This conclusion is supported by studies in experimental animals in which complete removal of one copy of these genes has no effect on lens transparency. Interestingly, mutations in crystallin genes are sometimes associated with microcornea. Since most of these genes have not been detected in the cornea, it appears that defects that originate in the lens lead to alterations in the size of the cornea.
Many of the transcription factors that promote the abundant expression of the crystallin genes in lens fiber cells have been identified. The details of these studies are described in comprehensive reviews and will not be considered further here.
Human lenses express two α-crystallin genes, αA and αB. Examination of the protein structure of the α-crystallins revealed that they are members of the widely distributed family of small heat shock proteins. An important function of small heat shock proteins is to stabilize proteins that are partially unfolded and prevent them from aggregating (this is termed their “chaperone” activity). The role of the α-crystallins in preventing protein aggregation and precipitation has been demonstrated in experiments performed in vitro. A similar, in vivo role for αA crystallin has been inferred in mice in which αA crystallin gene was disrupted. The function of the α-crystallins in preventing protein aggregation has obvious importance for the lens, because the proteins in lens fiber cells must persist for the life of the individual and excessive protein aggregation could lead to light scattering and cataract formation (see below).
Alpha-crystallins are also enzymes, since they possess serine-threonine auto-kinase activity. It is not yet clear whether the α-crystallins phosphorylate other proteins in lens fiber cells. The α-crystallins are, themselves, normally phosphorylated in vivo and can be phosphorylated in vitro in a cyclic AMP-dependent manner. , The factors that regulate the phosphorylation of α-crystallins and the importance of this phosphorylation for the function of the α-crystallins in the living lens have not been determined.
Alpha-crystallin proteins normally associate in the lens cell cytoplasm to make high-molecular-weight complexes containing approximately 30 subunits. The structure of these complexes has been revealed by cryoelectron microscopy. These observations show that native α-crystallin complexes can be assembled in a number of configurations, indicating that there is substantial flexibility in the way the subunits associate. Alpha-crystallin monomers also readily exchange between high-molecular-weight complexes, further supporting the view that α-crystallin complexes are quite plastic.
The phenotype of αA-crystallin knockout mice provides insight into the function of α-crystallins in vivo. The lenses of these animals are slightly smaller than normal, but structurally quite similar to the normal lens. Mature fiber cells contain aggregates of proteins that lead to the formation of cataracts, beginning a few weeks after birth. Analysis of these aggregates shows that they contain large amounts of αB crystallin and smaller amounts of other proteins. These results suggest that, in the fiber cell cytoplasm, αA crystallin is partly responsible for preventing αB from aggregating. In addition, when lens epithelial cells from these animals were cultured in vitro, they grew more slowly than normal cells, were more sensitive to stress, and had a higher rate of apoptosis, accounting for the smaller size of the knockout lenses. Therefore, αA crystallin appears to be important for the normal function of lens epithelial and fiber cells.
In addition to being expressed at very high levels in the lens, αB crystallin is present in a variety of cells throughout the body, especially in heart and skeletal muscle. The protein is also found in damaged areas of the central nervous system in a variety of neurodegenerative diseases, including in the “drusen” that accumulate at the basal surface of the retinal pigmented epithelial cells in age-related macular degeneration. A naturally occurring mutation in the αB crystallin gene (CRYAB) leads to the formation of cataracts and “desmin-related” myopathy. In vitro tests showed that the mutant form of the protein had no chaperone activity and even enhanced the aggregation of test proteins. These studies suggest that αB crystallin has important chaperone functions in the lens and in other cells of the body. However, the fact that the mutant protein accelerated the denaturation of test proteins leaves open the possibility that the mutant is a gain-of-function. In this case, the cataracts and myopathy seen in individuals harboring this mutation could be due to the destabilizing function of the protein, rather than loss of its function as a chaperone. However, additional mutations in CRYAB, which do not have myopathy as part of their phenotype, support the view that loss of its chaperone function contributes to cataract.
The β/γ crystallin superfamily is more diverse than the α-crystallins and the function of its members in the lens is less evident. The β- and γ-crystallins were originally thought to be two distinct protein families. However, once the protein sequences of representative members of these families were available, it was clear that they were closely related. The major difference in these proteins is the tendency for most of the β-crystallins to form multimers, while the γ-crystallins exist as monomers. Solving the three-dimensional structure of these molecules confirmed their close structural relationship. It also confirmed that the N- and C-terminal extensions of the β-crystallins provided a structural rationale for why these family members form higher-order aggregates. , There are six beta crystallin polypeptides (βA1, βA3, βA4, βB1, βB2, βB3), and three gamma crystallins (γS, γC, γD) expressed in the human lens, although the βA1 and βA3 polypeptides are derived from the same gene (βA3/A1). Beta- and gamma-crystallins bind calcium in vitro and may buffer this important cation in the lens fiber cell cytoplasm.
The lens fiber cell cytoskeleton
Microtubules are abundant beneath the plasma membranes of lens fiber cells where they probably play an important role in stabilizing the fiber cell membrane. Microtubules may also be important for transporting vesicles to the apical and basal ends of elongating fiber cells, although neither function has been demonstrated in vivo. In addition to microtubules, there is an abundant network of actin-containing microfilaments beneath the plasma membrane of lens fiber cells. These microfilaments associate with the cytoplasmic surfaces of the adhesive junctions between lens fibers and are also likely to interact with the spectrin-containing sub-membrane meshwork. , This sub-membrane scaffold also contains tropomyosin and tropomodulin, proteins that may modulate the structure of the microfilaments.
Lens fiber cells also contain an unusual complement of intermediate filaments, including those composed of vimentin. This is unusual, because vimentin intermediate filaments are usually restricted to cells of mesodermal, not epithelial origin. The function of vimentin-containing intermediate filaments in the lens is not evident, because mice lacking vimentin appear to have normal lenses. Over-expression of vimentin in the lens leads to cataract formation and defects in fiber cell differentiation. Whether this is a specific effect of excessive vimentin levels in the lens, or the response of the lens to protein over-expression in general, has not been demonstrated.
In addition to vimentin, the lens contains intermediate filaments composed of the proteins filensin and phakinin (see reviews , ). These filaments have an unusual “knobby” structure, leading to the name “beaded filaments.” Beaded filament proteins have only been found in lens fiber cells, suggesting that they have a specialized role in these cells. Mutations of both genes are associated with the formation of human cataracts, often involving the sutures. , Deletion of the phakinin gene ( Bfsp2 ) in mice caused a mild, progressive cataract that was associated with decreased levels of its binding partner, filensin, but no obvious defects in fiber cell differentiation.
Other cellular and biochemical specializations found in lens fiber cells
In fiber cells at early stages of elongation the lateral plasma membranes are smooth. However, as fiber cells reach the sutures, the membranes become progressively more interdigitated, forming interlocking “ball-and-socket” junctions ( Fig. 5.7 ). Ball-and-socket junctions may stabilize the lateral membranes of the fiber cells and assure that the cells remain tightly connected during accommodation. The mechanisms responsible for the formation of these unusual membrane specializations have not been identified.

The membranes of mature fiber cells have an unusual lipid composition. Human lens fiber cells have the highest proportion of cholesterol of any plasma membrane in the body and the amount of cholesterol increases as the fiber cells mature. , The cholesterol/phospholipid ratio of nuclear fiber cells is nearly three-fold greater than in cortical fiber cells. Partially purified fiber cell membranes have substantially lower cholesterol/phospholipid ratio than was found in the whole tissue, although membranes from nuclear fibers still have proportionally higher cholesterol content than membranes from the cortex. This finding suggests that some of the cholesterol in mature lens fiber cells may be associated with a complex that is not an integral component of the plasma membrane. There is also a high percentage of sphingomyelin in lens membrane phospholipids. The presence of high concentrations of cholesterol and sphingomyelin is likely to cause lens fiber cell membranes to be quite rigid. The functional significance of these biochemical specializations is not known.
In addition to its unusual lipid content, lens fiber cell plasma membranes contain several unique proteins. The most abundant of these is the “major intrinsic polypeptide” (MIP). MIP accounts for as much as 50 percent of the total protein of the lens fiber cell membrane and has not been detected in any other cells in the body. When the MIP cDNA was first cloned and sequenced it encoded a protein sequence that was unrelated to any known protein. Later, when the aquaporin family of water channel proteins was identified, MIP was recognized to be its “founding” member. In spite of its strong structural resemblance to the other aquaporins, MIP is a relatively inefficient water channel and may play a more important role in cell–cell adhesion. , Mutations in the MIP gene lead to cataracts in mice and humans. , These mutations could cause cataracts because they reduce MIP function, or because they interfere with the formation or function of lens fiber cell membranes.
The gap junctions of the lens are assembled from a unique set of subunits, or connexins. The cell-to-cell transport of small molecules (< 1 kDa) mediated by these gap junctions is likely to be important for the function of the lens, since most of the fiber cells are far from the nutrients supplied by the aqueous and vitreous humors. Not surprisingly, lens fiber cells have the highest concentration of gap junction plaques of any cells in the body ( Fig. 5.8 ).

There are three connexins found in lens cells, α1, α3, and α8. Connexin α1 (also known as Cx43) is found in many tissues in the body. In the lens, it is present only in the epithelial cells. Connexins α3 (Cx46) and α8 (Cx50) are abundant in fiber cells, but α8 is also expressed in lens epithelial cells. , In mice, epithelial α8-containing gap junctions are required for maximal proliferation of the epithelial cells in the first weeks of postnatal life. , Gap junction plaques containing both connexins α3 and α8 are present along the lateral membranes of lens fiber cells. Mutations in α3 or α8 are responsible for dominant congenital cataracts in several families.
While the presence of a large number of gap junctions would seem to be essential for the normal function of the lens, recent studies have shown that the lenses of mice lacking either the connexin α3 or α8 gene are relatively mildly affected. In the case of the α3 knockouts, fiber cell differentiation proceeds normally, although nuclear cataracts appear shortly after birth. However, no cataracts form when the disrupted α3 connexin gene is bred onto a different genetic background, indicating that genetic modifiers can compensate for the absence of this connexin in maintaining lens transparency. The lenses of the α8 knockouts develop diffuse nuclear cataracts. Considering the abundance of specialized connexins in the lens, these phenotypes seem surprisingly mild. Mouse lenses lacking α3 and α8 have more severe cataracts, suggesting that the functions of these proteins partially overlap.
A second pathway, which mediates the diffusion of larger molecules between lens cells has also been described. In chicken embryos, fiber cells fuse with their neighbors late in their differentiation, just before they degrade their organelles. Fiber cell fusions have been described in previous morphological studies in the lenses of several species. , Examination of fiber cell fusions in postnatal mouse lenses suggests that they mainly mediate the diffusion of proteins between fiber cells at a similar depth in the lens, rather than providing a diffusion pathway between more superficial and deeper fiber cells.
The second most abundant protein in the lens fiber cell membrane is MP20 (Lim2). The sequence of MP20 places in the tetraspanin family of proteins, with diverse functions in many cell types. Mutations in this gene lead to the formation of congenital cataracts in mice and humans. Knockout of Lim2 causes cataracts and defects in the lens refractive gradient. , The fiber cells in these lenses fail to fuse with their neighbors to establish the normal intercellular macromolecular diffusion pathway.
Lens fiber cells are linked to their neighbors all along their lateral membranes by N-cadherin, a calcium-dependent, homophilic cell adhesion molecule. , N-cadherin is typically linked to the actin cytoskeleton by a complex of proteins that includes α- and β-catenin. As the lens changes shape during accommodation, the lateral membranes of the fiber cells are held together tightly by interlocking ball-and-socket junctions and N-cadherin-containing cell–cell adhesion complexes. These adhesion complexes, along with Lim2, which has a role in cell–cell adhesion in the lens, are likely to contribute to the close association between the lateral membranes of lens fiber cells. Minimizing the extracellular space is important for reducing light scattering to maximize transparency.
In addition to the lateral cell adhesion complexes, lens fiber cells are joined tightly to each other at their apical and basal ends. These complexes contain abundant N-cadherin and vinculin, a protein that plays an important role in regulating the interaction between adhesion molecules and the actin cytoskeleton. Membrane complexes at the basal ends of the lens fiber cells, along the posterior capsule, probably attach the fiber cells to the capsule, since they contain a rich actin cytoskeleton, the contractile protein myosin, and integrin extracellular matrix receptors. , It is possible that the basal membrane complex also plays a role in the migration of the basal ends of the lens fiber cells towards the sutures.
The lens grows by progressively adding new layers of fiber cells to its outer surface. These new fiber cells elongate, gradually extending their apical and basal ends towards the sutures. Once the tips of an elongating fiber cell reach the sutures they meet a fiber cell coming from the opposite side of the lens. The cells then form new junctions at the apical and basal ends and detach from the posterior capsule (see Fig. 5.1 ). After they detach from the capsule, the fiber cells are gradually buried deeper within the lens by successive layers of “younger” fiber cells.
Soon after they detach from the posterior capsule, fiber cells suddenly degrade all of their membrane-bound organelles, including their mitochondria, endoplasmic reticulum, and nuclei. Organelle degradation is complete within a few hours. The mechanism responsible for organelle degradation in lens fiber cells is not known, although it has been suggested that the enzyme 15-lipoxygenase mediates organelle loss in the lens, as it does during the maturation of erythrocytes.
Numerous studies have pointed to the similarity between organelle degradation in the lens and the initiation of apoptosis (programmed cell death) in many other cell types. Although several biochemical similarities are striking, mice lacking the “executioner” caspases 3, 6 and 7 (which are critical mediators of apoptosis) showed normal organelle degradation. Knockout of the lysosomal enzyme, DNase IIβ, revealed that it plays a role in the removal of DNA fragments remaining after nuclear membrane fragmentation, but is not required for the initiation of organelle breakdown. Mice lacking the heat shock transcription factor, HSF4, have cataracts and failure of proper organelle loss, raising the possibility that HSF4 controls this process. ,
In primates, the outer shell of fiber cells that contain organelles is only about 100 microns wide. These organelle-containing cells are located, for the most part, outside of the optical axis of the lens (see Fig. 5.1 ). The absence of organelles in most lens fibers increases transparency by reducing light scattering. However, organelle loss also makes the fiber cells in the center of the lens dependent on the superficial fiber cells, an arrangement that may contribute to age-related cataract formation (see below).
The control of lens growth
The human lens grows rapidly in the embryo and during the first postnatal year. The rate of lens growth slows between ages 1 and 10, then continues at a much slower, nearly linear rate throughout life (see Fig. 5.5 ). , The factors that regulate lens growth in humans are not known. Many growth factors have been shown to stimulate lens epithelial cell proliferation in vitro and the receptors for several families of growth factors have been identified in the human lens and in the lenses of other species. These include the fibroblast growth factor family, the insulin-like growth factor family, the epidermal growth factor family, , platelet-derived growth factor, , hepatocyte growth factor, , and vascular endothelial cell growth factor. In addition to these growth factor receptors, there are a variety of other kinds of receptors, including muscarinic acetylcholine receptors and purinergic receptors. , It is not known whether any of these signaling systems is essential for lens growth in vivo. In this regard, deletion of EGF, PDGF, FGF or IGF receptors has not been associated with defects in lens growth or development. ,
Recent studies revealed an endogenous system regulating the growth of rodent lenses in vivo. The environment around the lens is severely hypoxic. When mice or rats breathed increased amounts of oxygen, oxygen levels increased in the eye. In older animals, oxygen treatment was associated with a more than three-fold increase in lens epithelial cell proliferation and long-term treatment led to greater lens wet weight. Younger lenses were not affected by oxygen levels and breathing lower oxygen did not alter lens growth at either age. These results suggest that the low oxygen levels that normally exist in the eye are required for the decline in lens growth that normally occurs with age. Subsequent studies showed that this decline probably required the oxygen-regulated transcription factor, HIF-1, since expressing oxygen-insensitive forms of HIF in lens epithelial cells suppressed the effect of oxygen on their rate of proliferation. Since lens size is an important risk factor for age-related cataracts, understanding how HIF-1 suppresses lens growth is likely to have clinical relevance.
Communication between lens epithelial and fiber cells
The apical ends of the lens epithelial cells abut the apical ends of elongating fiber cells. It has been suggested that nutrients are provided to the underlying fiber cells through gap junctions linking the apposed ends of these cells. Other studies have questioned this interpretation, since gap junctions are rarely detected at the apical ends of central epithelial cells. , Since the peripheral fiber cells contain a full complement of organelles, it is unclear whether transport from the epithelium is necessary for lens viability. The possibility that the lens does not require gap junction communication between the epithelial and fiber cells is supported by studies in which the main lens epithelial connexin, Cx43, was deleted in the lens and ciliary body. Although loss of this connexin in the ciliary epithelium reduced the production of aqueous humor, the lenses appeared normal. However, damage to lens epithelial cells compromises the viability of underlying lens fibers. Whether this is due to the absence of metabolites provided by the epithelium, or to some other role of the epithelium has not been determined.
Vascular support during lens development
Soon after it is formed, the lens becomes covered with a meshwork of capillaries. In the posterior of the lens this network, the tunica vasculosa lentis, arises from the hyaloid artery. The capillaries at the anterior of the lens arise from blood vessels of the developing iris stroma to form the anterior pupillary membrane. These capillary networks join with each other near the lens equator. It has been assumed the fetal vasculature is important for normal lens development, although similar vessels are never present around the lenses of non-mammalian species. Deletion of vascular endothelial growth factor (VEGF-A) from the lens prevented the formation of the fetal vasculature, resulting in smaller lenses with transient nuclear cataracts. Since mouse lens cells express functional VEGF receptors, further studies are required to determine whether these cataracts were due to loss of VEGF signaling to lens cells, to the absences of the fetal vasculature, or both.
During the second trimester of human development, the capillaries of the tunica vasculosa lentis and the anterior pupillary membrane regress. Decreasing levels of plasma-derived vascular endothelial cell growth factor may be one of the factors involved in the normal regression of these vessels. Macrophages in the vitreous body also appear to play an essential role in capillary regression. , In mice, these macrophages cause the programmed death of the endothelial cells by secreting the morphogen, Wnt7b.
A number of hereditary and acquired ocular diseases are accompanied by persistence of the fetal vasculature (see review ). At present, it is not clear why the fetal vasculature fails to regress in so many different syndromes and hereditary diseases. Better understanding of the factors that regulate vascular regression in normal ocular development is needed to address this question.
The lens as the organizer of the anterior segment
Studies performed in the 1960s and extended in the last decade show that the lens plays an important role in the development of the other tissues of the anterior segment. Absence of the lens at an early stage of embryogenesis leads to the absence of the corneal endothelium, abnormal differentiation of the corneal stroma, and absence of the iris, ciliary body, and anterior chamber. Therefore, the lens not only receives signals from its environment, but sends signals to nearby tissues that are essential for their normal development. The nature of these signals is unknown.
Special problems of lens cell metabolism
Overview
The lens, like all biological systems, is subjected to oxidative stress. Oxidation can be caused by molecular oxygen or free radicals. Oxygen free radicals are generated by the normal activity of mitochondria, by other metabolic processes, and by the absorption of light. To counteract the effects of oxidation, all cells maintain a reducing environment in their cytoplasm. The generation of reducing equivalents requires the expenditure of energy and, therefore, presents an especially difficult problem for the deeper lens fiber cells, which lack mitochondria. Enzyme systems in these deeper cells are also less active or inactive, since, in older lenses, they would have been synthesized decades earlier. For this reason, central fiber cells maintain a precarious balance between the catastrophic damage that would be caused by the unchecked oxidation of membrane lipids and cytoplasmic proteins and the diffusion from the more superficial cells in the lens of molecules that protect against oxidative damage. ,
The unique structure of the lens creates special problems for the majority of fiber cells that do not contact the lens epithelium or capsule. Nutrients must reach these cells by diffusion, either between cells or through specialized cell–cell junctions. To reduce light scattering and maintain transparency, lens fiber cells must maintain a very small extracellular space. Therefore, nutrient and metabolite transport is more likely to occur through cells, rather than between them. This predicts that metabolites will accumulate in the center of the lens and that diffusion will limit the availability of nutrients and essential metabolites to cells deeper in the lens. Mature fiber cells do not synthesize proteins and must deal with the consequences of molecular senescence without the capacity for repair.
The lens derives much of its energy from glycolysis. , The end product of glycolysis is lactic acid. As a result of lactate accumulation, intracellular pH drops significantly from peripheral to deeper fiber cells. , As a result, pH-sensitive processes will be differentially affected in different regions of the lens. Gap junction conductance is one of the systems in the lens that could be affected, since gap junction permeability is generally decreased at low pH. Interestingly, lens connexins do not decrease their conductance in response to the decreased pH in the mature fiber cells of the mouse lens. , This adaptation preserves a diffusion pathway from the lens periphery to its center. Other structural and metabolic adaptations to the standing pH gradient in the lens are likely to exist.
Another problem that the lens faces is the need to maintain protein stability for many decades. Once the lens is formed, proteins are synthesized only in the superficial fiber cells. Therefore, proteins made during embryogenesis in humans may have to last for more than 100 years. Accumulated damage leads to loss of enzymatic activity. Altering the structure of the crystallins, cytoskeletal proteins, and enzymes also increases their propensity for aggregation, a process that can lead to cataract formation.
Oxidants within and around the lens
All of the cells of the body exist in an oxidizing environment. Molecular oxygen is, directly or indirectly, the source of most oxidative damage. If cells could survive in an atmosphere free of oxygen, most oxidative damage could be avoided. For most cells this is not possible. However, human lenses can be maintained in an atmosphere of pure nitrogen for some time, as long as adequate amounts of glucose are provided. This is possible because the lens obtains much of its energy from glycolysis. ,
The oxygen tension around the lens in the living eye is quite low, <15 mmHg (~2% O 2 ) just anterior to the lens and <9 mmHg (~1.3% O 2 ) near its posterior surface. Oxygen levels within the human lens are even lower (<2 mmHg). The low oxygen tension around and within the lens helps to protect lens proteins and lipids from oxidative damage. Even with this low level of oxygen, the lens normally derives a proportion of its ATP from oxidative phosphorylation, a process that, of necessity, generates free radicals.
Hydrogen peroxide is another molecule that has been suggested to cause oxidative stress to the lens. Hydrogen peroxide is produced in mitochondria by the enzyme superoxide dismutase acting on superoxide anion, a byproduct of oxidative phosphorylation. Hydrogen peroxide can also be produced during the oxidation of ascorbic acid, which is present at high levels in the aqueous and vitreous humors (approximately 1.5–2.5 mM). Both processes may contribute to the hydrogen peroxide that has been reported within the intraocular fluids and lens. , The level of hydrogen peroxide in the aqueous humor has been reported to average over 30 µM and to exceed 200 µM in about one-third of cataract patients. , However, recent studies have suggested that, for methodological reasons, the level of hydrogen peroxide in aqueous humor was overestimated in these earlier studies. Ascorbate interferes with some assays of hydrogen peroxide and aqueous humor produces hydrogen peroxide when exposed to air. , Careful re-examination of the levels of hydrogen peroxide around and within the human lens using methods not subject to these errors is needed.
The lens is exposed to solar irradiation throughout its life. Although the most energetic and potentially harmful ultraviolet light that reaches the eye is absorbed by the cornea, the remaining solar radiation could have harmful effects, especially on the metabolically vulnerable fiber cells. If light is not absorbed, it produces no damage. However, ultraviolet light is readily absorbed by a number of cellular constituents, including DNA, proteins, nucleoside-containing metabolites, flavinoids and pigments. Flavinoids and pigments also absorb visible light, especially the shorter wavelengths of visible light. All of these interactions are potential sources of free radicals. Free radicals oxidize DNA, lipids, and proteins. Whether or not free radicals are produced by the absorption of light depends upon the chemical nature of the molecule that is interacting with light and the molecular environment.
In spite of its exposure to light throughout life and the presence of abundant targets for light damage, there is no evidence for the photo-oxidation of proteins in the central region of the lens, even in lenses from older individuals. , It is likely that lens constituents are protected against the harmful effects of light-generated free radicals by the high intracellular concentration of reducing substances (see below), and by the low concentration of oxygen around and within the lens.
Protection against oxidative damage
Glutathione, a tripeptide of the amino acids glutamine, cysteine, and glycine, provides most of the protection against oxidative damage in the lens. It can prevent the oxidation of components of the lens cytoplasm because its concentration in the lens is very high, approximately 4–6 mM, and its sulfhydryl group is readily oxidized. When glutathione levels have been lowered in lens epithelial cells or whole lenses, cell damage and cataract formation follow rapidly.
Lens epithelial and superficial fiber cells can synthesize glutathione and glutathione can be transported into lens from the aqueous humor. Reduced glutathione (GSH) is regenerated from oxidized glutathione (GSSG) by glutathione reductase and NADPH ( Fig. 5.9 ). Much of the NADPH in the lens is produced by the hexose monophosphate shunt, the activity of which is important for the continued production of reduced glutathione.

However, fiber cells deeper in the lens have minimal capacity for the synthesis or reduction of glutathione. They must obtain most of their reduced glutathione by diffusion from more superficial fiber cells. , Glutathione can form disulfide bonds with the oxidized sulfhydryl groups of proteins. These glutathione-protein mixed disulfides can then be reduced by a second molecule of glutathione, a process that is facilitated by the enzyme thioltransferase. This regenerates the protein sulfhydryl and forms GSSG (two molecules of glutathione linked by a disulfide bond). The GSSG that results from this process must then diffuse to more superficial layers of the lens where it can be reduced to regenerate GSH ( Fig. 5.10 ). It is likely that this two-way diffusion is the rate-limiting step in maintaining a reducing environment in the center of the lens. , The rate of diffusion between the superficial and deeper layers of the lens diminishes with age. Therefore, proteins and lipids in the nuclei of older lenses may be more susceptible to oxidative injury than those in younger lenses.

Ascorbic acid is also likely to protect the lens against oxidative damage. Ascorbate is actively transported from the blood to the aqueous humor by a sodium-dependent transporter located in the ciliary epithelium and reaches concentrations in the aqueous humor that are 40–50 times higher than levels in the blood. The ascorbate levels in the lens and other intraocular tissues are also substantial. , Dehydroascorbate, the oxidized form of ascorbic acid, enters lens cells by way of the glucose transporter, where it is reduced by glutathione-dependent processes. , Like glutathione, ascorbate is readily oxidized, forming dehydroascorbic acid in the process. Therefore, ascorbate can react with free radicals and other oxidants in the aqueous humor and the lens, preventing these molecules from damaging lens lipids, proteins, and nucleic acids. On the other hand, if dehydroascorbate accumulates in the lens, its metabolites can react with lens proteins, increasing lens color and decreasing protein stability. The high GSH levels in the lens are likely to maintain most ascorbate in its reduced state, thereby avoiding much of this potential damage.
The lens has two enzyme systems to detoxify hydrogen peroxide. Lens epithelial cells have abundant levels of catalase, which converts hydrogen peroxide to water, and glutathione peroxidase, an enzyme that couples the reduction of hydrogen peroxide to the oxidation of glutathione. Studies on cultured lenses and lens epithelial cells suggest that glutathione peroxidase provides most of the protection against the potential damaging effects of physiological levels of hydrogen peroxide, as catalase is only effective against relatively high concentrations of peroxide.
Energy production in the lens
Due to the lack of blood supply, the oxygen concentration within and around the lens is much lower than in most other parts of the body. The lens, therefore, depends on glycolytic metabolism to produce much of the ATP and the reducing equivalents required for its metabolic activities. , The glucose required for glycolytic metabolism is derived from the aqueous humor. Aqueous humor glucose levels are maintained by facilitated diffusion across the ciliary epithelium.
However, lens epithelial cells and superficial fiber cells also contain mitochondria. Therefore, cells near the surface of the lens use both glycolytic and oxidative pathways to derive energy from glucose. About 50 percent of the ATP produced by rabbit lens epithelial cells is derived from oxidative metabolism, while glycolysis accounts for nearly all the ATP produced in lens fiber cells.
Water and electrolyte balance
Due to its high protein concentration and the lack of a blood supply, the lens faces special problems in regulating its water content and in providing nutrients and antioxidants to cells deeper in the lens. Protein concentration increases as one goes from the more superficial fiber cells to fiber cells deeper in the lens. However, this protein gradient is not associated with a reciprocal gradient in the osmotic activity of water, because there is no tendency of water to flow into the cells of the lens nucleus. The mechanism by which this large potential osmotic gradient is neutralized is not known. Decreased protein osmotic activity might result from the increase in the short-range interactions between protein molecules that contributes to lens transparency. The mechanism by which proteins can be concentrated in osmotically neutral manner remains an important unsolved question in the biophysics of the lens.
Several studies provide evidence for an ionic circulation within and around the lens. Vibrating electrodes detect gradients of electrical potential around the lens. , Positive charges flow into the lens near the anterior and posterior sutures and out at the equator. Like most cells, the membranes of lens epithelial and superficial fiber cells contain sodium, potassium-activated ATPase activity. This Na + , K + -ATPase generates an electrochemical gradient across the surface membranes of the lens, with the interior of the lens more negative than the extracellular space. The electrochemical potential tends to drive positive charges, largely sodium ions, into lens cells. This appears to be the origin of the inward positive current at the sutures. The positive current flowing out at the lens equator is likely to be carried by potassium ions.
A model that takes into account the electrical and biophysical properties of the lens predicted that water will follow the flow of ions into and out of the lens, creating an internal circulation system. While this is an appealing theory, direct evidence for water flow through the lens fiber cell cytoplasm has not been provided. Numerous studies in which fluorescent dyes were injected into fiber cells have, so far, failed to detect the directed, bulk movement of water that would be predicted by this model. Other studies detected inward flow of water across the lens epithelium and the whole lens, suggesting net flow of water from the anterior chamber to the vitreous body. The importance to lens physiology of this potential flow has not been tested.
The transmembrane potential of the human lens decreases steadily with age. This decrease is caused by an increase in the permeability of the fiber cell membranes to sodium and calcium ions through non-selective cation channels. It is not clear whether the increased cation permeability is due to increased numbers of these channels, to the appearance of the new type of channel, or to an increase in the activity (open probability) of pre-existing channels. The increase in cation permeability is balanced by an increase in the activity of membrane ATPases, which remove sodium and calcium from lens cells. In spite of the increased pump activity, free sodium and calcium levels increase in the cytoplasm of older lenses. Since the transmembrane potential of all cells indirectly provides the driving force for the transport of many metabolites and nutrients, the age-related decrease in the transmembrane potential of the lens may have important consequences for lens metabolism and ionic homeostasis. Elevated calcium levels can also lead to metabolic disturbances and destruction of cell components through the activation of calcium-sensitive proteases.
Like all cells, lens epithelial and fiber cells maintain a much lower concentration of free calcium ions in their cytoplasm than is found in the extracellular space. However, free calcium levels measured in fiber cell cytoplasm are substantially higher than the levels in epithelial cells. , In addition, there appears to be a gradient of free calcium that decreases from the posterior to the anterior ends of lens fiber cells. These observations are consistent with the view that calcium slowly leaks into lens fiber cells and is removed by membrane pumps at the lens surface. The activity of these pumps in maintaining low cytoplasm calcium concentrations is important, because treatments that abruptly raise free calcium levels lead to rapid degradation of the lens fiber cell cytoskeleton, uncontrolled proteolysis, cell swelling, and opacification.
Lens epithelial cells transport nutrients into their cytoplasm from the aqueous humor. Although the transport of small molecules from epithelial cells to fiber cells has been demonstrated, , the relative importance of this pathway in providing nutrients to the fiber cells, compared to transport directly across the surface membranes of superficial fiber cells, has not been determined. When metabolite transporters have been examined in lens fiber cells, these molecules have usually been found. The distribution of glucose transporters in the lens illustrates this issue. Lens epithelial cells express abundant levels of the glucose transporter, glut1, that is presumably used for the uptake of glucose from the aqueous humor. Although fiber cells express little glut1, they express large amounts of the higher-affinity glucose transporter, glut3. Therefore, fiber cells can transport glucose into their cytoplasm from the extracellular milieu, a finding that raises questions about the relative importance of the epithelial cells in providing glucose to the fiber cells.
Lens transparency and refraction
Vertebrate lenses are remarkably effective optical devices. An efficient lens must be transparent to the wavelengths of light that can be detected by the photoreceptors, have a focal point that is appropriate for the optical system in which it functions, and have a minimum of spherical and chromatic aberration. Lens transparency depends on the organization of the cells of the lens and of the distribution of the proteins within them. The precise organization of the fiber cells, their high protein concentration, and the absence of organelles from the fiber cells that lie in the optical axis assures that a minimum of scattering occurs as light passes through the lens.
The cellular and molecular interactions responsible for establishing and maintaining the curvature of the lens surfaces are not known. Studies in chicken embryos demonstrated that influences from outside the lens normally regulate its shape and rate of growth. Lenses in different species range from nearly spherical (rodents) to an axial ratio of more than 2:1 (humans). As the human lens grows, the radii of curvature of its anterior and posterior surfaces decrease significantly. In spite of this, the focal point of the lens remains remarkably constant, suggesting that the refractive power of the lens cytoplasm changes in a way that compensates for the change in curvature of the refractive surfaces. Control of lens shape is one of the most fascinating unanswered (and little explored) aspects of lens biology.
The high protein concentration of the lens fiber cells causes the refractive index of the lens to be higher than that of the fluid around it. Fiber cells close to the surface of the lens have a lower protein concentration than fiber cells deeper in the lens, creating a gradient of refractive index that at least partially corrects for spherical aberration.
Although the human lens is transparent to most wavelengths of visible light, it produces and accumulates chromophores that absorb the shortest wavelengths of the visible spectrum. At birth, the human lens is a pale yellow color. With increasing age the amount of yellow pigmentation in the lens increases. This pigmentation absorbs the shorter, more energetic wavelengths of light, preventing them from reaching the retina. The predominant yellow chromophores in the young human lens are metabolites of tryptophan, especially N-formyl kynurenine glucoside. With aging, an increasing variety of soluble and protein-bound chromophores are found in the lens fiber cells. , When especially high concentrations of these chromophores accumulate, they can reduce visual acuity by increasing light absorbance, leading to the formation of what is termed a “brunescent” cataract. The factors responsible for the excessive accumulation of chromophores in some lenses are not known, although recent studies have suggested that the oxidative modification of proteins may play a significant role. Brunescent cataract is more common in rural areas and in developing countries, a factor that suggests that nutritional or environmental factors, like cooking smoke, may also be important.
Changes in the lens with aging
Fiber cells in the center of an adult lens were produced during early embryonic life, while those at the surface of the lens may be weeks or months old. Many have touted the lens as a valuable model system for studying aging. However, unique properties of the lens, especially the lens fibers, make it an inappropriate model for aging in most cell types. Gene expression and protein synthesis cease at about the time that the fiber cells degrade their organelles. In nearly all other cell types, RNA and protein synthesis and turnover continue throughout life.
To follow changes in the composition of the lens that are related to age, one must correlate changes in fiber cells from lenses of different ages and from different layers of the same lens. Using this approach it was shown that, in the human lens, many of the soluble crystallins are sequentially truncated by proteolysis over a period of months to years. Some of the β-crystallins are degraded relatively rapidly, while other crystallins much more slowly. However, by the end of the first 20 years of life, most of the crystallins appear to reach a steady state after which little additional degradation occurs. Therefore, in older lenses, crystallins in the center of the lens and crystallins a short distance from the surface of the lens show similar degrees of proteolysis. These studies only examined changes in soluble crystallins. Further studies may show that crystallins in the insoluble fraction are more extensively degraded.
The proteins of the lens are often characterized by their relative solubility, typically being separated into fractions that are water-soluble, urea-soluble, and “insoluble”. As one goes from more superficial to deeper fiber cells, an increasing percentage of the crystallins are found in the insoluble fraction. In addition, when one separates the water-soluble crystallins by size, an increasing proportion is found in high molecular aggregates. Therefore, there is a general tendency for proteins to become aggregated and less soluble in the older fiber cells. , Alpha-crystallin is a good example. In the lens nucleus from individuals of increasing age, one finds decreasing amounts of soluble α-crystallin. By age 45, no α-crystallin is detectable in the water-soluble fraction from the lens nucleus. ,
The gradual insolubilization of α-crystallin is likely to be related to its function as a molecular chaperone. When lens proteins are partially unfolded, hydrophobic domains are exposed. Alpha-crystallin binds to these hydrophobic regions, presumably preventing uncontrolled protein aggregation. It seems likely that accumulated damage to the crystallins and other proteins leads to increased association with α-crystallin. Interestingly, in spite of the loss of soluble α-crystallin, one does not see a precipitous increase in the rate of protein aggregation in the lens nucleus after age 45.
Another age-related change seen in crystallin structure is the increasing racemization of aspartic acid, methionine and tyrosine, and the deamidation of glutamine and asparagine. Racemization and deamidation alter protein structure and/or charge. These changes correlate well with age, but do not differ significantly in clear and cataractous lenses of the same age.
Some components of the lens fiber cell cytoskeleton are disassembled in older fiber cells. Vimentin intermediate filaments are degraded in the deeper lens cortex, well after the loss of membrane-bound organelles. Beaded filaments composed of phakinin and filensin persist into the lens nucleus and may last for the life of the lens. Actin microfilaments also appear to survive in the oldest fiber cells, although the persistence and continued association of these filaments with the plasma membrane has been questioned. Proteolysis and insolubilization of the components of the cytoskeleton appear to contribute to their disassembly.
The structure and development of the lens sutures
Sutures form at the anterior and posterior poles of the lens where fiber cells growing from opposite sides of the lens abut at their apical and basal ends. In some species all fiber cells meet near the midline of the lens, forming an “umbilical” suture. In most species, the sutures form along planes. In human embryos, elongated lens fiber cells meet at three planes, forming an upright “Y” at their anterior ends (with respect to the superior–inferior axis of the eye) and an inverted “Y” posteriorly ( Fig. 5.11A ). As the human lens grows, the suture planes formed by more superficial shells of fibers become increasingly complex. The first evidence of this typically occurs soon after birth, when two new suture planes form at the ends of each of the three branches of the Y sutures ( Fig. 5.11B ). As new fibers are added during lens growth, the branch points of the newly formed sutures gradually “migrate” towards the center, eventually forming a six-pointed “star” suture ( Fig. 5.11C ). Branching again occurs at the tips of each of these six planes, eventually forming a total of 12 suture planes at the anterior and posterior surfaces of the lens ( Fig. 5.11D, E ). The increasing geometric complexity of the suture patterns in older human lenses results in lenses with better optical properties than in species that maintain a simple Y suture pattern throughout life.

The formation and orientation of the sutures provides a challenging problem for developmental, cell, structural and theoretical biologists. The lens appears to be radially symmetrical about the optical axis. Yet, the sutures form in a pattern that breaks this symmetry. The spatial cues that lead to the precise alignment of the suture planes with respect to the axes of the eye and body are not known.
The lens capsule
When the lens placode invaginates from the surface ectoderm early in embryonic life, it is already supported by a thin basal lamina. This extracellular matrix surrounds the lens vesicle after it detaches from the surface ectoderm. The lens epithelial and superficial fiber cells continue to secrete the components of the basal lamina, which thickens to become the lens capsule. , Examination of the lens capsule in the electron microscope shows that it is composed of multiple laminae, as if the basal lamina had been replicated many times. Studies of the synthesis of the lens capsule in experimental animals showed that newly synthesized capsular materials are originally deposited close to the basal ends of epithelial and fiber cells. With time, the labeled components of the capsule move farther away from the surface of the cells, as they are displaced by successive layers of newly synthesized capsular material.
Clinical observations have also supported the view that the human lens capsule is synthesized from the inside out. In certain cases, foreign materials may be deposited in the capsule during its synthesis. These deposits can be viewed in slit lamp or Scheimpflug images. Eventually, these deposits disappear from the lens. One interpretation of this observation is that, as new layers are laid down on the inner surface of the capsule, capsular material is lost from the surface of the lens. If this interpretation is correct, the rate of synthesis of components of the capsule at its inner surface and the rate of degradation at its outer surface regulate its thickness. The capsule also must be remodeled during embryonic life, when the surface area of the lens is increasing rapidly. The enzymes that are responsible for the degradation or remodeling of the capsule have not been identified.
In keeping with its similarity to typical basal laminae, the lens capsule is composed predominantly of type IV collagen, laminin, entactin (nidogen), and heparan sulfate proteoglycans. However, studies have shown that some components of the capsule differ in the anterior, posterior, and equatorial regions of the lens. In the adult lens the capsule is significantly thicker over the epithelium and thinner over the basal ends of the superficial fiber cells. The factors that regulate the differential distribution and accumulation of the components of the lens capsule remain to be identified. Several diseases are associated with defects in the components of the capsule, including pseudoexfoliation syndrome (lysyl oxidase polymorphisms), Pierson syndrome (laminin-β2 mutations) and Alport syndrome (collagen IV mutations).
The zonules
The zonules (suspensory ligament, zonules of Zinn) are composed of thin fibrils that suspend the lens in the anterior of the eye. Zonular fibers insert into the lens capsule near the equator and into the basal lamina of the non-pigmented layer of the ciliary epithelium ( Fig. 5.12 ). It is likely that the ciliary epithelial cells synthesize the components of these fibrils. The primary structural protein in zonular fibers is fibrillin. Mutations in the fibrillin gene are responsible for Marfan syndrome, in which dislocation of the lens is a common clinical finding. ,

Examination of the insertion of zonular fibers into the lens capsule shows that these fibers are intimately interwoven with the components of the capsule. If, as suggested above, the capsule is continuously degraded at its outer surface, it is unclear how the zonular fibers and capsular fibers maintain their connections.
This is only one of the topological paradoxes associated with the synthesis and maintenance of the zonules. For example, it is also not clear how the components of the fibrils are originally inserted into the lens capsule, especially when they are under tension, or how the zonular fibers are physically attached to the basal lamina of the ciliary epithelial cells while being synthesized by these same cells. Presumably, the zonular fibers establish their attachment between the lens capsule and ciliary epithelium early in development when these basal laminae are in direct contact. If this assumption is correct, it would predict that the number of zonular fibers should not change throughout life. If it is not correct, a mechanism must exist for these fibrils to be assembled across the space between the ciliary epithelium and the lens capsule, a remarkable feat of bioengineering.
As the lens grows, the position of insertion of the zonules shifts anteriorly. Since the zonules are believed to maintain a fixed point of insertion into the lens capsule, this shift has been interpreted as evidence for a relative increase in capsule synthesis at the lens equator. It is possible that the age-related anterior shift in the zonular insertion point alters the forces applied on the lens during accommodation and may contribute to presbyopia.
Cataracts
A cataract is any opacification of the lens. Cataracts are considered to be clinically significant when opacification interferes with visual function. Loss of lens transparency can be due to an increase in light scattering or light absorption. Increased light scattering can be caused by disruption of the structure of lens fiber cells, increases in protein aggregation, phase separation in the lens cell cytoplasm, or by a combination of these processes.
Age-related cataracts are classified based on the region of the lens that is affected ( Fig. 5.13 ). The most common types of age-related cataracts are nuclear, cortical, and posterior subcapsular. Nuclear cataracts occur in the oldest fiber cells near the center of the adult lens – those formed during embryonic and fetal life. Cortical cataracts occur in cells formed later in life. These cataracts typically begin in a sector of the lens cortex, affecting mature cells that have already degraded their organelles. Posterior subcapsular cataracts result from light scattering by a plaque of swollen cells at the posterior pole of the lens.

In addition to age-related cataracts, there is a wide variety of less common cataracts that are usually classified based on their etiology. These include the opacification of the posterior lens capsule that sometimes occurs following cataract surgery, referred to as secondary cataracts, after cataracts, or posterior capsular opacification (PCO).
Cataract epidemiology
The risk factors associated with different types of age-related cataracts provide clues to the environmental factors that promote cataracts or protect against them. Therefore, before considering the mechanisms that may cause age-related cataracts, it is useful to consider the factors that influence the risk of cataracts in human populations. Although epidemiologic factors are often difficult to identify with certainty, especially for a disease that occurs late in life, those factors that are frequently associated with cataracts in human populations are likely to be relevant to the disease mechanism.
General risk factors
Not surprisingly, age is the primary risk factor for the most common kinds of cataract. There is an exponentially increasing incidence of cataract after age 50. One risk factor common to most kinds of cataract is lower socioeconomic status and/or lower education. Since lower socioeconomic status may predispose to nutritional deficiencies, increased exposure to diseases, poor general health status, and increased occupational exposure to cataractogenic agents, it is difficult to determine the specific aspects of lower socioeconomic status that are important for cataract formation.
Sex is also an important influence on the incidence of cataract. Women are at increased risk for most kinds of cataract. Conversely, studies have suggested that estrogen protects against cataract formation in humans and animals and that cataracts may be delayed by late menopause. Lowering estrogen function with the anti-estrogen tamoxifen increased the risk of cataracts when used for long duration. , The protective effect of estrogen suggested by these studies makes the increased overall levels of cataract in women harder to understand. If estrogen is protective, other factors, as yet unknown, must strongly predispose women to cataract formation. Alternatively, higher levels of male sex steroids may be protective against cataract.
When the influences of age, sex and socioeconomic status are removed, specific risks for different types of cataracts are revealed. Smoking and high alcohol consumption have been identified in several studies as dose-dependent risk factors for nuclear and, in some cases, cortical cataracts. Dark iris color is often associated with a higher incidence of all types of lens opacities, a finding that may also be related to higher levels of cortical cataract in blacks than whites. Exposure to anti-inflammatory steroids and ionizing radiation are well-recognized risks for posterior subcapsular cataracts. Few additional risk factors have been consistently identified for PSCs. However, this could be the statistical consequence of the generally lower frequency of PSCs than of other types of cataract. Numerous epidemiological studies have identified additional risk factors for cataracts. These are not detailed here, either because their association with cataract was not especially strong, or because they have not been seen in the majority of epidemiological studies of cataract.
Several studies have found an interesting association between cataracts and increased mortality. When confounding variables, including smoking, age, race, and sex were removed, the presence of clinically significant cataracts remained as a strong, independent predictor of mortality, especially in younger patients with cataract. These findings suggest a connection between cataracts and systemic phenomena influencing survival. Considered in another way, behavioral, nutritional and biochemical factors that prevent age-related cataract formation may lengthen life span. However, other studies failed to find an association between cataract and increased mortality. , A recent 5-year follow-up of patients undergoing cataract surgery in the UK in 2000–2001 revealed lower mortality than in the general population. This paper also provides a thorough compilation of previous studies of cataract and mortality.
Several studies found an association between lens thickness and the most common types of age-related cataract. In the Beaver Dam Eye Study, a comprehensive evaluation of ocular disease in a US population of northern European ancestry, cortical cataracts correlated with thinner and nuclear cataracts with thicker lenses. Five-year follow-up of subjects that initially had clear lenses revealed that having a thinner lens was a risk for developing cortical cataract. Thicker lenses at baseline were associated with increased risk of developing nuclear cataract. Studies in Singapore and India subsequently found similar associations between lens thickness and age-related cataract. , Possible reasons for these associations are discussed below in the sections on nuclear and cortical cataracts.
Age-related nuclear cataracts
In the US and most Western countries, nuclear cataracts are most often associated with increased light scattering in the nuclear fiber cells. In many developing countries brunescent nuclear cataracts are more common. These opacities are associated with increased lens color and light absorption. Whether due to light scattering or absorption, nuclear cataract is the most common type in most countries or regions, typically accounting for more than 60 percent of cataract surgery. However, cortical cataracts predominate in some countries or regions, particularly in Asian populations living in temperate climates.
There is abundant evidence that nuclear cataracts are associated with increased oxidative damage to lens proteins and lipids. The formation of disulfide bonds between protein subunits can lead to aggregation and increased light scattering, although other forms of protein–protein interactions also occur. There is also evidence that crystallins may associate with lens fiber cell membranes in increased amount in nuclear cataracts. The factors responsible for increased oxidation in nuclear cataracts are not fully understood. However the marked age dependence of nuclear cataracts and the concurrent increase in oxidized glutathione in the lens nucleus suggests that the balance between protein and lipid oxidation and glutathione-dependent reduction are likely to be involved (see Box 5.3 ) .
Nuclear cataract is associated with the oxidative damage to the proteins and lipids, leading to hardening of the lens nucleus and increased light scattering. Hardening of the lens nucleus increases its refractive power, causing a “myopic shift” which is later followed by cataract formation. The lens normally exists in an extremely hypoxic environment. Patients treated with long-term hyperbaric oxygen therapy develop a myopic shift and, eventually, nuclear cataracts. Removal of the vitreous body during retinal surgery increases oxygen levels at the posterior of the lens and leads to rapid-onset nuclear cataracts. Retina surgery performed without vitrectomy avoids cataract formation. The high level of ascorbate (vitamin C) that is present in the ocular fluids reacts with oxygen, reducing oxygen exposure to the lens. Age-related degeneration of the vitreous body is associated with lower ascorbate levels, slower oxygen consumption and increased risk of nuclear cataract. Protecting the lens from exposure to oxygen or preserving the gel structure of the vitreous body could prevent the formation of nuclear cataracts.
Persuasive evidence for an association between oxidation, age, and cataract formation was provided by studies of patients treated with hyperbaric oxygen to alleviate the complications of peripheral vascular disease. These individuals were exposed to 2.0–2.5 atmospheres of pure oxygen for one hour each day for up to three years. Of the 25 patients treated in this study, all but one had increased light scatter in the lens nucleus and nearly half developed frank nuclear cataracts. All of the individuals who showed increased light scattering or opacification in the nucleus were over 50 years old. The individual who did not show increased light scatter was 24 years old. Patients of slightly older average age with the same presenting symptoms, but not treated with hyperbaric oxygen, served as the control group. None of the individuals in the control group developed cataracts over the study period. After the termination of treatment, some of the patients who had been treated with hyperbaric oxygen and had developed increased light scatter showed improvements in their visual acuity and decreases in the amount of light scattering in their lenses. This result suggests that, even in older individuals, the lens has the capacity to reverse oxidative damage to the components of the nucleus.
The onset of nuclear cataract formation is often associated with an increase in the refractive power of the lens. For hyperopic patients this “myopic shift” temporarily causes an improvement in their near visual acuity, a phenomenon that is often called “second sight.” This increase in refractive power is associated with hardening of the lens nucleus. It is not surprising that the term “nuclear sclerotic cataract” is used to describe the opacities that soon follow.
Patients treated with hyperbaric oxygen also experience significant myopic shift. As with the light scattering described above, these increases in lens refractive power usually reversed during treatment or soon thereafter. This suggests that the hardening of the lens and its increased refractive power, as occurs in nuclear sclerotic cataracts, are caused by oxidative damage to the proteins and lipids of central lens fiber cells. In this case, reducing the source of oxidative damage (hyperbaric oxygen) permitted the lens to recover much of its normal refractive properties.
Isolated lenses and experimental animals have also been treated with hyperbaric oxygen. The results of these studies are in general agreement with the findings in patients receiving hyperbaric oxygen therapy, although frank nuclear opacities are rarely seen. These animal models provide useful tools to study the earlier biochemical effects of oxidative stress on cataract formation.
Studies of patients treated with hyperbaric oxygen suggest that molecular oxygen or a metabolite derived from molecular oxygen contributes to nuclear sclerosis and nuclear cataract formation. Studies of the effect of hyperbaric oxygen on the lens also provide some of the strongest evidence that the cells of the lens nucleus are in a delicate balance between their ability to prevent or reverse oxidative damage and the tendency for oxidation to occur. The fact that hyperbaric oxygen leads to opacification of the lens nucleus, but not the more superficial lens fiber cells, shows that the central fibers are more susceptible to oxidative damage, even though they are farther away from the source of oxygen. Age-associated decrease in the rate of diffusion of glutathione to the lens nucleus is likely to be part of the reason for this increased susceptibility.
Nuclear cataracts occur frequently in older patients within 6 months to 3 years after vitrectomy. Several studies have reported that the incidence of cataracts is as high as 95% 2 years after the removal of the vitreous body. Evidence is accumulating which suggests that study of post-vitrectomy cataracts may help to understand the general mechanism responsible for the formation of age-related nuclear cataracts.
The appearance of the lens in post-vitrectomy nuclear cataracts is similar to typical age-related nuclear cataracts, the only difference being the rapidity of onset of cataracts after vitrectomy. If one assumes that the etiology of the cataracts in both cases is the same, there is likely to be an increase in oxidative damage in the lens following vitrectomy. The lens is usually not touched during vitrectomy and the time between surgery and cataract formation is at least several months. Therefore, the high incidence of nuclear cataracts after this procedure is probably due to changes in the environment around the lens, not to direct damage to the lens during surgery. Measurement of oxygen near the lens after vitrectomy showed that oxygen levels increased greatly during surgery and remained significantly elevated in the eyes of patients who returned for a second retinal surgery months or years later. When retinal surgery could be performed without damaging the vitreous gel, cataract was prevented, even 5 years after the initial surgery. In a related study, eye bank eyes with a greater degree of vitreous degeneration (liquefaction) were more likely to have nuclear opacities than eyes with a more intact vitreous gel. In eyes between 50 and 70, the extent of vitreous liquefaction was a stronger predictor of nuclear opacification than age. Diabetics, who have lower levels of oxygen in the vitreous, also progress to cataract surgery more slowly after vitrectomy than non-diabetics. These studies suggest that the vitreous gel protects against nuclear cataract, probably by protecting the lens from exposure to oxygen from the retinal vasculature.
The high concentration of ascorbate in the vitreous body also appears to protect the lens from exposure to oxygen. There is usually ~2.0 mM ascorbate in the human vitreous. , Degeneration of the vitreous body or prior vitrectomy was associated with a ~40 percent decrease in the mean ascorbate concentration. An endogenous catalyst enabled the ascorbate in the vitreous to react with oxygen. Presumably, ascorbate-dependent oxygen consumption reduces the exposure of the lens to oxygen. When the level of ascorbate or the catalyst in the vitreous fluid decreased, the ability of the vitreous to consume oxygen also decreased. Therefore, excessive vitreous degeneration or vitrectomy lowers the concentration of ascorbate in the vitreous, which would be expected to increase the exposure of the lens to oxygen ( Fig. 5.14 ).
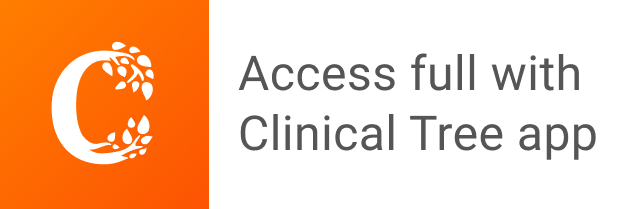