Introduction
The retinal pigment epithelium (RPE) is a monolayer of pigmented cells located between the light-sensitive photoreceptor outer segments and the fenestrated endothelium of the choriocapillaris. On both sides, specialized extracellular matrices enable a close interaction of the RPE with its adjacent tissues. On the basolateral side, the multilayered Bruch’s membrane combines barrier function and selective transport matrix as part of the blood retina barrier. On the apical side of the RPE, the interphotoreceptor matrix provides an interface for interaction between the photoreceptor outer segments and the RPE. In humans, every RPE cell interacts with a mean of 23 photoreceptors with variations between macular and peripheral cells. Through these interactions, the RPE forms a functional unit with the photoreceptors ( Fig. 13.1 ). The formation of this functional unit affects embryonic development, with the RPE and neural retina depending on each other for their differentiation, as well as for maturation and maintenance. This is evidenced by the effect of mutations in genes expressed in the RPE that lead to primary photoreceptor degeneration and mutations in genes expressed in photoreceptors that lead to primary RPE degenerations. As an integral component of this functional unit the RPE fulfills a multitude of tasks that are essential for visual function. The failure of any one of these functions leads to retinal degeneration.

Absorption of light
The pigmented RPE covers the inner wall of the bulbus and absorbs scattered light to improve optical quality by removing stray photons of light. In the human eye, light is focused by a lens onto the central part of the retina (macula) leading to accumulation of potentially excessive high-energy light that is absorbed by melanin granules within melanosomes in the RPE protecting the photoreceptors. This causes a temperature increase in the RPE that is then dissipated by choroidal blood flow. The relative blood perfusion of the choriocapillaris is very high, higher than that of the kidney. , Despite this, only small amounts of oxygen are extracted by the adjacent tissues and venous blood from the choroid, which has an oxygen saturation of more than 90%. This functional arrangement produces an excess of oxygen in combination with a large density of light energy, leading to a risk of photo-oxidative damage owing to production of reactive oxygen species. , As a consequence, the RPE is protected by various lines of defense against this toxicity. In addition to melanin in the RPE, there are carotenoids, lutein, and zeaxanthin, in the neural retina that absorb light energy. In addition, ascorbate, α-tocopherol and β-carotene, and glutathione are nonenzymatic antioxidants, and melanin itself can function as an antioxidant. This is further augmented by the natural ability of RPE cells to repair damaged DNA, lipids, and proteins.
Transepithelial transport
The RPE forms the outer blood-retinal barrier. The RPE is, by its electrophysiological parameters, a tight epithelium in which the paracellular resistance is at least 10 times larger than that of the transcellular resistance. , This barrier is formed by two types of connections on the lateral domains of adjacent RPE, apical zonulae occludens (tight junctions) and zonulae adherentes (adherens junctions), which form a barrier that regulates transepithelial diffusion through the paracellular spaces. This barrier function is also important for the immune privilege of the eye (see further in chapter). Owing to this tight barrier function, the complete exchange of molecules and ions between the blood stream and the photoreceptor side relies on transepithelial transport through the RPE.
Transport from the blood side to the photoreceptor side
The transport from the blood to the photoreceptor side consists mainly of transport of nutrients such as glucose, omega-3 (ω3) fatty acids, and retinal.
For glucose transport, the RPE contains an abundance of the glucose transporters GLUT1 and GLUT3 in both the apical and the basolateral membrane. , GLUT3 mediates the basal transport, whereas GLUT1 is responsible for inducible glucose transport that adapts to different metabolic demands.
All-trans retinol (vitamin A) is taken up from the blood stream via a receptor-mediated process involving a serum retinol binding protein/transthyretin complex. Retinol is converted to all-trans retinyl ester and then directly enters the visual cycle (see further in chapter).
Docosahexaenoic acid (DHA) is a polyunsaturated ω-3 fatty acid (22:6ω3) that is a major structural lipid of retinal photoreceptor outer segment membranes and is essential for outer segment renewal, but it cannot be synthesized by the photoreceptors. DHA is synthesized in the liver from the precursor linolenic acid and transported to the eye in the blood bound to plasma proteins. The RPE preferentially takes up DHA via the sodium-dependent lyso-phosphatidylcholine transporter, Mfsd2a, in a concentration-dependent manner.
Transport from the retinal side to the blood side
The retina is one of the most energy-demanding tissues, due primarily to the photoreceptors that are among the most metabolically active cells. This results in the production of large amounts of water and accumulation of lactic acid. Additional water is moved toward the retina by intraocular pressure from the vitreous. Water cannot pass through the RPE via the paracellular route because the RPE is a tight epithelium. Therefore, water and lactic acid are eliminated from the subretinal space by active transcellular transport by the RPE.
The transport of water is driven by an active transport of Cl − from the retina though the RPE to the blood side ( Fig. 13.2 ). , The energy for this process is supplied by the apically located Na + /K + -ATPase, which uses ATP to transport Na + out of the RPE in exchange for transport of K + into the cytosol of the RPE. The K + ions are recycled across the apical membrane through inward rectifier potassium channels, which provide the large K + -conductance of the apical membrane. This recycling keeps the K + gradient across the apical membrane small to support the activity of the Na + /K + -ATPase. On the apical membrane a Na + /2Cl − /K + -cotransporter uses the Na + gradient across the apical membrane to transport K + and Cl − into the cytosol of the RPE. Since K + moves back through inward rectifier channels to the subretinal space, the Na + /2Cl − /K + -cotransporter accumulates Cl − in the intracellular space of the RPE cells (40–60 mM). This high Cl − concentration provides a driving force for Cl − to leave the cell. The basolateral membrane displays a large conductance for Cl − through which Cl − leaves the cell from the intracellular space to the blood side. This is the last step of transepithelial Cl − transport from the subretinal space to the blood side, resulting in a basolateral negative transepithelial potential between –6 and –15 mV. Voltage-gated ClC-2 Cl − channels appear to provide a major part of the basolateral Cl − conductance. Other channels providing basolateral Cl − conductance are Cl − channels linked to intracellular second-messenger systems. Cl − transport can be increased by rises in intracellular free Ca 2+ or by increases in cytosolic cAMP concentration resulting from either activation of Ca 2+ -dependent Cl − channels or cAMP-dependently regulated Cl − channels.

Large subretinal concentrations of lactic acid are formed by the high metabolic activity of the retina and removed by the RPE ( Fig. 13.2B ). , This lactic acid transport requires a tight regulation of the intracellular pH. , Lactic acid is removed from the subretinal space by the monocarboxylate transporter 1 (MCT1), which transports H + in cotransport with lactic acid (in concentrations that can be as high as 19 mM). This transport is driven by the activity of the Na + /H + -exchanger that eliminates H + from cytosol of the RPE cells using the gradient for Na + provided by the activity of the Na + /K + -ATPase. Across the basolateral membrane, lactic acid leaves the cell via the activity of another monocarboxylate transporter, MCT3, which is also an H + /lactic acid cotransporter. Intracellular pH is stabilized by the transport of bicarbonate (HCO 3 − ). , HCO 3 − is transported into the cell across the apical membrane by the Na + /2HCO 3 − -cotransporter which uses the Na + gradient established by the Na + /K + -ATPase. HCO 3 − leaves the RPE cell across the basolateral membrane by the activity of the Cl − /HCO3 − exchanger.
Production of metabolic water and accumulation of lactic acid are linked. Therefore, transport of water and pH regulation are also coupled. An increase in the transport of lactic acid leads to intracellular acidification that inhibits the transport activity of the Cl − /HCO3 − exchanger. Since the Cl − /HCO3 − exchanger transports Cl − in the opposite direction from the Cl − channels, it decreases the Cl − transport efficiency in resting conditions. The inhibition of the Cl − /HCO3 − exchanger, thus, increases the transepithelial transport of Cl − and water. This is further increased by the ClC-2 channels, which are activated by a decrease in extracellular pH. Extracellular acidification can result from the increased transport of lactic acid by the MCT3 in the basolateral membrane ( Box 13.1 ).
Retinal detachment is characterized by a separation of the retinal pigment epithelium (RPE) from the photoreceptor layer of the neuroretina. This separation prevents close interaction between the two layers, leading to reduction of visual function. After successful closure of the retinal break, preventing access of fluid from the vitreous to the subretinal space, remaining subretinal fluid reabsorbs spontaneously. The electrolyte and fluid pump of the RPE is responsible for the elimination of subretinal fluid.
RPE cells are postmitotic and thus do not usually divide, but under certain circumstances they may become activated to play a role in wound repair. These wound repair mechanisms can also have devastating consequences by creating so-called “proliferative vitreoretinopathy” (PVR). RPE cells migrating through retinal tears in the vitreous cavity and on the retinal surface may transdifferentiate into myofibroblasts, creating contracting membranes that cause complicated retinal traction detachments that are difficult to repair.
Capacitive compensation of fast changes in the ion composition in the subretinal space
In the dark, cGMP-gated cation channels in photoreceptor outer segments generate an inward current of Na + and Ca 2+ that is counterbalanced by a K + outward current at the inner segments. In the light, cGMP-gated cation channels close and the K + current is decreased, resulting in a decrease of the K + concentration in the subretinal space from 5 to 2 mM. The decrease of the Na + conductance in the light in outer segments leads to an increase in the subretinal Na + concentration. In addition, illumination of the retina also causes an increase in the subretinal volume. Both light-induced changes are compensated for the moment they occur, by modulation of ion transport by the RPE. ,
Compensation for changes in the K + concentration and in extracellular volume are both linked to the potassium transport. The apical membrane of the RPE displays a large K + conductance. Decrease in the subretinal K + concentration leads to hyperpolarization of the apical membrane that corresponds to the c-wave in the electroretinogram. The decrease in the potassium concentration decreases the activity of the Na + /K + /2Cl − -cotransporter, which subsequently decreases the intracellular Cl − activity and hyperpolarizes the basolateral membrane. On an electroretinogram this can be seen as the delayed hyperpolarization. The hyperpolarization of the apical membrane activates inward rectifier K + channels that generate an efflux of K + into the subretinal space to compensate for the light-induced decrease in the subretinal K + concentration. At the same time, the apical hyperpolarization decreases the activity of the Na + /HCO3 − -cotransporter that is an electrogenic transporter by its stoichiometry. The subsequent intracellular acidification increases the transepithelial Cl − and water transport as described earlier.
The light-induced increase in subretinal Na + concentration is compensated for by the activity of the Na + /K + /2Cl − -cotransporter and by the Na + /H + exchanger. The Na + /K + -ATPase takes up Na + to compensate for the increase in the subretinal Na + concentration during the transition from light to dark. This task appears to be the reason for its localization in the apical membrane of the RPE.
Visual cycle
The visual cycle is initiated in photoreceptor outer segments by a photon reacting with rhodopsin, consisting of a G-coupled receptor opsin (rods) or cone opsin (cones) and the light-detecting chromophore, 11-cis retinal, which is photo-isomerized to all-trans retinal. To maintain visual function, regeneration of 11-cis retinal from all-trans retinal occurs through a complex process initiated in the photoreceptors that is completed in the RPE ( Fig. 13.3 ). , All-trans retinal leaves the disc membrane as N-retinylidene-phosphatidylethanolamine (N-retinylidene-PE), the Schiff base adduct of retinal and PE, by the importer activity of the ATP-binding cassette protein (ABC) transporter, ABCA4 (also known as ABCR or the rim protein), , which flips N-retinylidene-PE across the photoreceptor disc membrane. Following dissociation from N-retinylidene-PE, all-trans retinal is reduced to all-trans retinol by retinol dehydrogenase (RDH). All-trans retinol diffuses across the interphotoreceptor matrix chaperoned by the interphotoreceptor retinoid binding protein (IRBP) into the RPE. , In the cytosol of the RPE, it binds to the cellular retinol binding protein (CRBP). All-trans retinol is esterified by lecithin:retinol transferase (LRAT) to all-trans retinyl esters. These may be stored as retinosomes or converted to 11-cis retinol by RPE65, and oxidized by 11-cis retinol dehydrogenase (11cRDH or RDH5) back to 11-cis retinal.chaperoned by cellular retinaldehyde binding protein (CRALBP). The 11-cis retinal diffuses across the IPM with IRBP into photoreceptor outer segments where it completes the cycle by recombining with opsin. Cones have an additional cone-specific visual cycle involving Müller cells.

Since the visual cycle maintains the excitability of the photoreceptors, it needs to be adapted to the different photoreceptor activities between light and dark. In the light, there is a fast turnover of retinal, whereas in the dark the turnover occurs much more slowly. Retinal that is not needed in the state of a slower turnover must be quickly available for the transition from dark to light, and vice versa. The different retinal binding proteins represent connected pools for retinal. In the transition from dark to light, IRPB represents the first pool that delivers retinal. This pool cannot be depleted because it refills retinal from the pool of CRALBP proteins. CRALBP in turn can recruit retinal from RPE65. Thus, with increasing light intensity retinal can be recruited from the different pools, and in the transition from light to darkness retinal can be stored. IRBP can carry larger amounts of retinal in the dark than in the light. Another key function is the modulation of the RPE65 function. In the light, RPE65 is palmitoylated and bound to intracellular membranes. In this configuration, RPE65 predominantly catalyzes the re-isomerization of all-trans retinol to 11-cis retinol. In the dark, RPE65 is water soluble and freely diffusible in the cytosol and in this configuration, RPE65 may function as a retinal store, although there is some question about the physiologic relevance of the form ( Box 13.2 ).
The regeneration of the visual pigment in the retinal pigment epithelium (RPE) involves a complex cascade of steps and a variety of molecules. Mutations in these molecules can cause retinal diseases. Mutations in RPE65 can cause the severe congenital blinding disease Leber’s congenital amaurosis (LCA). Mutations in the RLBP1 gene and its product, CRALBP, have been identified in patients with autosomal recessive retinitis punctata albescens, a tape to retinal degeneration.
Clinically these diseases manifest with different degrees of visual loss. They include congenital blindness (LCA), and slow loss of central vision with peripheral visual field remaining intact throughout life (Stargardt’s disease). Other forms are night blindness with progressive loss of the peripheral visual field progressing to blindness at an adult age. Some are forms of retinitis pigmentosa, a class of diseases involving progressive degeneration of the retina, typically starting in the midperiphery and advancing toward the macula and fovea. The first proof-of-concept gene augmentation therapy was for the treatment of LCA caused by RPE65 mutations and was performed in a dog model. This led to the first US Food and Drug Administration (FDA) approval of a gene therapy, voretigene neparvovec-rzyl (Luxturna), for an inherited disease, in 2017.
Phagocytosis of photoreceptor outer segments
The focus of high levels of light energy onto the retina and increased formation of reactive oxygen species by photo-oxidation constantly expose photoreceptor outer segments to toxic compounds, leading to outer segment damage. To maintain the excitability of the photoreceptors, outer segment discs are renewed at the connecting cilium every day , as evaginations of the ciliary membrane. The damaged distal tips of the photoreceptor outer segments are shed and phagocytosed by the RPE through interactions with the interdigitated apical RPE microvilli. The process of outer segment disc renewal and shedding and phagocytosis by the RPE is tightly coordinated to maintain the proper length of the outer segments. Coordination of phagocytosis is a receptor-mediated multistep process leading to the efficient degradation of the internalized photoreceptor outer segments (POS). Rod outer segment disc shedding is regulated in a circadian manner at light onset. , Every 11 days the equivalent of a whole length of a photoreceptor outer segment is renewed
Rod outer segment phagocytosis by the RPE consists of recognition, binding, internalization, and degradation of the ingested outer segment ( Fig. 13.4 ). The first step in phagocytosis is recognition, a receptor-mediated process involving the integrin αvβ5, which interacts with phosphatidylserine of rod OS (outer segement) plasma membranes. This binding initiates a signal cascade in the RPE involving the activation of focal adhesion kinase (FAK). This process serves two functions. One leads to phosphorylation of the TAM receptor tyrosine kinase, MerTK, another receptor that is involved in the regulation of phagocytosis. The second function leads to the circadian regulation of phagocytosis. MerTK triggers the process of internalization of POS after binding to v-integrin. Protein S and Gas6 both function as bona fide TAM receptor ligands in vivo. The downstream cascade initiated after activation of MerTK involves the generation of inositol-1,4,5-trisphosphate and an increase of cytosolic free Ca 2+ as second messenger. The ligand, CD36, is also required to activate the OS internalization process of the RPE ( Box 13.3 ).
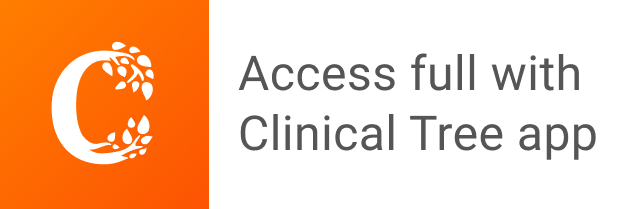