The Bookend Technique and Other Scaling Techniques
Jessy J. Mouannes-Srour
Timothy J. Carroll
Introduction
In this chapter, the quantification of cerebral perfusion by rescaling the relative values by an appropriate calibration constant to produce meaningful quantitative values for cerebral blood volume (CBV, mL/100 g), cerebral blood flow CBF (ml/100 g/min), and mean transit time (MTT, seconds) is discussed. Relative values are derived from the first pass of a bolus of contrast agent using dynamic susceptibility-contrast (DSC)–weighted perfusion images.1,2,3,4 In the seminal papers of Ostergaard et al.5,6 on the deconvolution analysis, the authors found that gray-to-white matter perfusion ratios in DSC perfusion images were consistent with those obtained in H2[O15] positron emission tomography (PET) measurement of perfusion. Because PET is a widely recognized standard of reference, this leads to the conclusion that, if a proper scaling factor can be determined, magnetic resonance imaging (MRI) perfusion values can be calibrated (i.e., rescaled) to yield accurate quantitative perfusion values. In this chapter, evidence is presented to support this hypothesis and a number of rescaling methods are introduced. “The bookend method,” which has had some success in this regard, is discussed in detail.
Perfusion in Acute Stroke
In a setting of acute ischemic stroke, the threshold for perfusion below which tissue becomes infarcted depends on both the degree of hypoperfusion (i.e., reduced CBF) and its duration. The areas that have been subjected to prolonged hypoperfusion can be identified as diffusion positive (i.e., hyperintense) on MRI scans. In acute stroke, the time from symptom onset defines the duration parameter, but the absolute level of perfusion is difficult to determine with MRI. This has prompted the American Heart Association to recommend the following: “More research must be conducted to make these techniques, especially the MR-based methods, quantifiable.”7
Chemical thrombolysis has been shown to significantly reduce neurologic deficits resulting from stroke.8,9,10,11,12,13 However, treatment is currently limited to a window of 3 hours after onset of symptoms. Recombinant tissue plasminogen activator (rtPA), the only U.S. Food and Drug Administration (FDA)–approved pharmacologic therapy, is administered intravenously during this time window.11 This time restriction limits the rate of intervention to a mere 1% to 2% of patients who present with acute ischemic stroke.10,12 The treatment poses a substantial (10% to 15%) risk of hemorrhage.
The DEFUSE 2 investigators13 demonstrated that MRI scans where a perfusion–diffusion mismatch is evident can identify patient subgroups that will benefit from therapies that lead to reperfusion, during extended time windows, that is, 3 to 6 hours after the onset of symptoms. Consequently, patients who are beyond the 3-hour time limit and are currently ineligible for treatment may possess large amounts of hypoperfused, yet salvageable, brain tissue. In such patients, thrombolysis has been shown to be beneficial for 6 to 24 hours after the onset of initial symptoms.14 Furthermore, patients with severe hypoperfusion have a higher risk of cerebral hemorrhage within the 3-hour time limit.15
Subacute Disease: The Stages of Hemodynamic Failure
The presence of neurovascular disease in the intracranial circulation or in the extracranial carotid and vertebral arteries disrupts the normal delivery of oxygen to the brain. The degree of compromise is normally referred to as hemodynamic failure, and its quantification has been the subject of many imaging studies. Of particular interest is determining the stages of hemodynamic failure, since more severe failure is associated with a higher risk of stroke.16,17 In fact, owing to the wide range of normal anatomic variants in the intracerebral vascular anatomy (presence or absence of anterior and posterior cerebral arteries), observation of the hemodynamic compromise is believed to be a better predictor of stroke risk than the degrees of stenosis in vascular disease.
The stage of hemodynamic failure can be determined as alteration in the normal CBV, CBF, and cerebrovascular reserve (CVR, the percentage change in CBF under physiologic challenge), and, most importantly, the increase in the brain’s oxygen extraction fraction (OEF).18,19 Figure 27.1 shows a graphical representation of these changes. Stage 1 is characterized by normal CBF and increased CBV resulting from reflex vasodilation to maintain normal flow. In stage 2, hemodynamic failure (also known as “misery perfusion”), the vasodilatory response is maximal and CBF begins to fall, whereas OEF increases to maintain the normal metabolic rate of oxygen metabolism. In Nemoto’s model depicted in Figure 27.1, cerebrovascular reserve, decreases nearly linearly, with compromise,
and may potentially be used to identify the stage of hemodynamic failure, which has been shown to predict future strokes.20 The interplay of all these parameters, with the potential of predicting an impending stroke, indicates the need for robust quantification of parameters related to cerebral perfusion.
and may potentially be used to identify the stage of hemodynamic failure, which has been shown to predict future strokes.20 The interplay of all these parameters, with the potential of predicting an impending stroke, indicates the need for robust quantification of parameters related to cerebral perfusion.
![]() FIGURE 27.1. Changes in cerebral blood volume (CBV), cerebral blood flow (CBF), and oxygen extraction fraction (OEF) can be used to quantify hemodynamic failure in intracranial vascular disease.19 The quantification of perfusion parameters will be used to identify chronic hemodynamic compromise in longitudinal study of patients to identify those in need of intervention. Ipsi, ipsilateral; Contra, contralateral. |
Dynamic Susceptibility Contrast Magnetic Resonance Imaging
In a DSC-MRI measurement of cerebral perfusion, a time-series of T2– or T2*-weighted echo-planar images (EPIs) is acquired in conjunction with an intravenous bolus injection of a paramagnetic contrast agent. Contrast agent concentration is calculated by first converting the signal intensity to the contrast agent concentration:

where C(t) is the contrast agent concentration in tissue at time t after injection, S(t) is the corresponding signal intensity at time t, S0 is the preinjection signal, TE is the echo time, and K reflects the contrast agent relaxivity and properties of the pulse sequence. C(t), commonly known as the “tissue-concentration curve,” is used to calculate the CBV and CBF. The relative CBV (rCBV) in the DSC-MRI measurement is given by:

where AIF(t) is the tissue-concentration curve measured in or near an artery, ρ is the density of brain tissue, and HLV and HSV are the hematocrit values of the large and small vessels, respectively.21 The relative CBF (rCBF) is calculated through deconvolution of the tissue concentration curve C(t) and the arterial input function (AIF(t))5, according to:

where R(t) is the residue function.
Rescaling Based on Population-Averaged Flow
Ostergaard et al.6 proposed rescaling rCBF of subcortical white matter (WM) to the normative value of 22 mL/100 g/min as a mean of estimating whole brain perfusion. Given the ability and physiologic need of the brain to autoregulate toward normative values, this approach gives an excellent approximation for normal values, and it can be of great value in a setting of acute stroke where complex image acquisition strategies or lengthy postprocessing steps are contraindicated. Bristow et al.22 have shown that this population-averaged approach to rescaling can produce clinically useful images of CBF, and the potential to predict the ischemic thresholds for cell death. However, the population-averaged flow values ignore the well-known age dependence of normal perfusion, which has been shown to decrease by 5% to 7% per decade in WM and 7% to 10% in gray matter (GM), as measured in PET and MRI experiments.23,24 Furthermore, this approach ignores protective vasodilation and cannot be used in a setting where intracranial pressures are managed pharmacologically, as in intracranial hemorrhage.
Rescaling Relative to External Reference Values
Ostergaard et al.25 first proposed the rescaling of MRI values to PET to test the hypothesis that rescaling of MRI perfusion values would yield agreement with reference standard PET values. They performed a study of cerebral perfusion in six normal pigs using both MRI and PET scans. They determined an empirical rescaling factor,
Φ= 1.09, through a correlation analysis of MRI and PET perfusion values. When rescaling the MRI values by this common scaling value, they found a good correlation between PET-rescaled MRI values and reference standard PET values. In that study, the corrected flow values correlated well with H2[O15] PET values measured in the same subjects (slope = 1.1, r = 0.86).
Φ= 1.09, through a correlation analysis of MRI and PET perfusion values. When rescaling the MRI values by this common scaling value, they found a good correlation between PET-rescaled MRI values and reference standard PET values. In that study, the corrected flow values correlated well with H2[O15] PET values measured in the same subjects (slope = 1.1, r = 0.86).
![]() FIGURE 27.2. 26(A) Performing a global rescaling of magnetic resonance imaging (MRI) perfusion values by assigning a cerebral blood flow (CBF) value of subcortical white matter showed poor correlation (r = 0.6/0.56) for perfusion ipsilateral/contralateral to a carotid artery occlusion. (B) Mukherjee et al.26 have found that MRI CBF values (i.e., perfusion), if properly rescaled, correlate well with standard of reference positron emission tomography (PET) perfusion values. |
Mukherjee et al.26 studied 11 patients with unilateral carotid artery occlusion. They performed DSC perfusion MRI and H2[O15] PET perfusion in these subjects and compared the CBF values obtained from MRI to those obtained from PET, which served as a reference standard for CBF. They estimated the effect of bolus delay and dispersion by comparing perfusion values with Arterial Input Function (AIF) chosen in the middle cerebral artery ipsilateral and contralateral to the carotid occlusion. In addition, they determined the applicability of calibrating subcortical WM to the population averaged value.6,22,23
To determine the validity of using a global calibration factor to rescale the DSC-MRI CBF values, they rescaled the MRI to the “true” perfusion values measured with PET. They compared the resulting MRI and PET values using correlation analysis (Fig. 27.2). Mukherjee et al.26 found that MRI CBF values, if properly rescaled, correlate well with standard of reference values. A global rescaling of MRI perfusion values undertaken by assigning a CBF value of subcortical WM showed poor correlation (r = 0.5/0.56) for perfusion ipsilateral and contralateral to a carotid artery occlusion.
Furthermore, in patients with a significant delay in the arrival of the bolus relative to the normal side, these authors found significant differences in the measured MRI perfusion values. The AIF results underscore the need for more advanced deconvolution analysis techniques, such as circular deconvolution27 or delay and dispersion-corrected local AIFs,28 but lead to the conclusion that rescaling relative perfusion values by an appropriate scale factor may be a means to produce quantitative perfusion values from MRI.
Rescaling Based on Venous Outflow
Lin et al.29 proposed using in MRI perfusion images, an internal reference that would provide quantitative perfusion values without the need for PET images. The internal reference chosen was the integrated venous output function (VOF). They derived a correction factor by dividing the integrated VOF, measured in a region of interest (ROI) covering a large vein, by the average VOF values acquired in volunteers. They found significant improvement in the patient-to-patient variability when relative perfusion values were corrected by the VOF methods. In a group of five volunteers and five patients with angiographically confirmed carotid artery occlusion, MRI CBF values were highly correlated with those measured by PET in the same subjects (slope = 1.02, r = 0.8). Figure 27.3 shows representative perfusion images from Lin et al.29 and the dramatic improvement in the correlation between MRI and PET CBF that the proposed VOF rescaling provides.
Although the VOF results were encouraging, the method assumes the following: (a) The injected dose for each subject is fixed, (b) the fraction of the cardiac output that goes to the brain is constant, and (c) signal nonlinearities in the VOF measurement are identical. Furthermore, the VOF in a patient was normalized to that of the average VOF in a group of volunteers, so, in a sense, the VOF measurement is also a population-averaged technique.
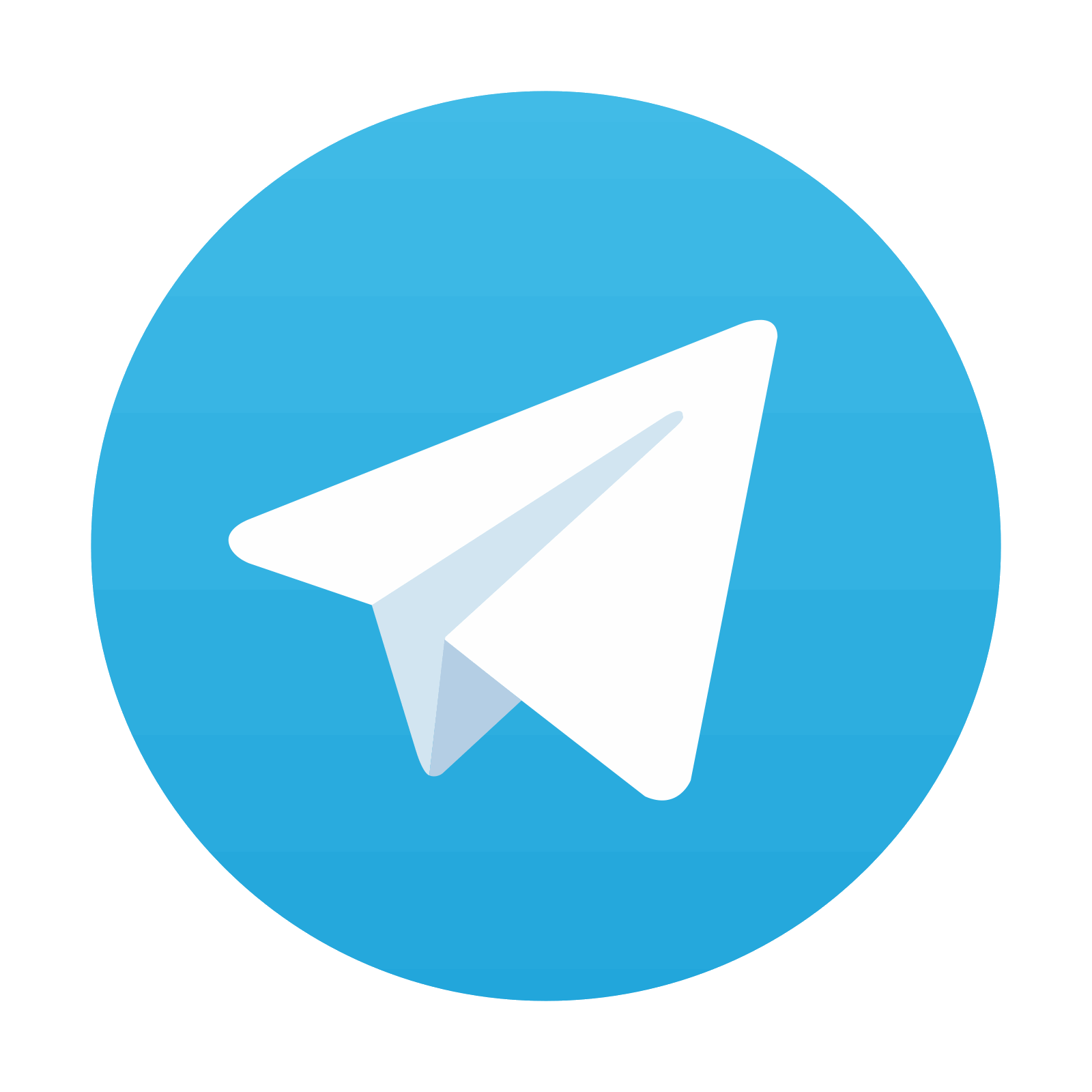
Stay updated, free articles. Join our Telegram channel
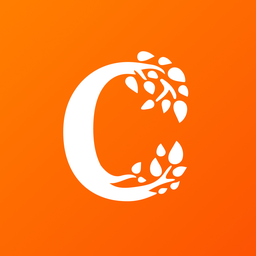
Full access? Get Clinical Tree
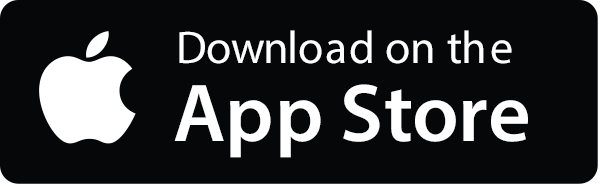
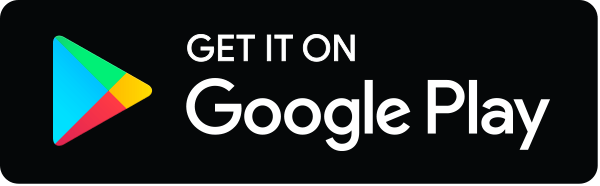