Technological Advances in Rhinology and Anterior Skull Base Surgery
Summary
The technological progress in endoscopy and radiology of the last few decades created the foundation for a refined pathophysiologic understanding and better differentiated surgical treatment in rhinology. Since the first pioneering experiments with instrument guidance, endoscopy, powered instruments, and robots, today′s commercially available technical systems no longer remind the user of the highly experimental and artisanal prototypes. Contemporary, widely available technology can be used by ordinary surgeons without a cohort of engineers and technicians. This chapter not only gives an overview of the well-established technical tools, but also presents a limited selection of promising new inventions and research instruments that still have to stand the test of time.
Computer-assisted Surgery
Terminology
The term Computer-Assisted Surgery (CAS) was defined around 1990 by Georg Schlöndorff and his group, who described it as a “new navigation aid for skull base surgery.”1 It introduced the idea of displaying the spatial position of a tool in relation to patient anatomy (medical image) on a computer screen. Since then, different terms have been used to describe this technique and have eventually evolved to precisely describe important development aspects, instrument guidance systems (IGSs), and surgical navigation. One fundamental component of every IGS is the hardware used to measure the spatial position of an instrument in relation to patient anatomy, which we will refer to with the term tracking system or position sensor.
History
Before the development of three-dimensional (3D) imaging techniques such as computed tomography (CT) and magnetic resonance imaging (MRI), stereotactic instrument guidance was mainly achieved through mechanical guidance and template mechanisms typically targeting structures in the brain. In 1987 Watanabe et al published the development of a frameless 3D digitizing system, called the Neuronavigator.2 Unlike modern systems, this approach deployed a mechanical coordinate measuring system that was essentially a robotic arm without electrical drives. This mechanical arm measured spatial positions and orientations very accurately and transferred this information to a computer for further processing, such as visualizing the instrument′s position relative to the patient′s anatomy. Similarly, the first CAS navigation system dedicated especially for endoscopic sinus surgery (ESS) developed by a group at Aachen University incorporated a mechanical measuring arm.3 Eventually, mechanical measurement devices used in IGSs were replaced by optical tracking. Further advancements through extensive academic research and industrial development allowed computer-assisted instrument guidance to become widely accepted as a standard of care for surgical procedures, especially around the head. A great variety of dedicated technological solutions have been applied to many different clinical application areas in the past 2 decades, such as in neurosurgery, ear, nose, and throat (ENT) procedures, and craniomaxillofacial (CMF) surgery, as well as in orthopedic and trauma surgery.
Technology Overview
Imaging
Technical progress in imaging techniques has paralleled the refinements and advancements in “disease-tailored” endonasal sinus surgery to embody what we now call functional endoscopic sinus surgery (FESS). Thus, the choice of imaging modality is dictated by the pathology to be treated. For simple chronic rhinosinusitis (CRS) and uncomplicated revision surgery, (low-dose) CT and digital volume tomography (DVT) data will suffice. On the other hand, surgery for invasive tumors, vascular anomalies, or infectious complications require high-dose and contrast-enhanced CT, MRI, or a combination of both providing optimal resolution for soft tissue invasion and serving as the basis of precise soft tissue navigation. In special cases, invasive arterial or venous angiography, MR angiography,4 intrathecal cisternography, scintigraphy, and even positron emission tomography (PET) are adjunctive examination techniques used to clear the nature or surgical resectability of disease if conventional imaging techniques are ambivalent. Finally, imaging is especially important in the application of IGS or CAS, which is rapidly growing in FESS, skull base surgery, and otological surgery for safety, didactic, and medicolegal reasons.5
Adequate and effective imaging is extremely important for proper diagnosis and treatment. For instance, elective imaging should be performed only after maximal topical and antibiotic therapies have been attempted to document residual disease that cannot be further treated without surgery. Although FESS with MR-based IGS is feasible, preoperative acquisition of CT-based datasets for navigation is still standard.
Care should be taken to ensure that images are acquired in a manner that satisfies region of interest (ROI), slice thickness, and image resolution requirements. For example, datasets intended for IGS not only should include the roof of the frontal or bottom of the maxillary sinus but also must adequately depict the contour of the face (tip of the nose, upper teeth, etc.) to allow surface matching (registration) of the data. Generally, axial CT sections 0.5 to 1 mm thick are needed to allow adequate coronal and parasagittal reconstructions.6 Today′s imaging resolution reaches pixel and voxel sizes in the millimeter (MRI) and submillimeter (CT) range, which is rarely insufficient. In our experience over the past 5 years, failure to use CT datasets by referring radiologic suites was most often due to inadequate image boundaries (ROI), followed by image slice thickness, and poor resolution occurring in 57, 29, and 14% of rejections, respectively (unpublished data).
Current CT technology provides the surgeon with detailed and excellent (often excessive) picture quality. However, this detailed information has an associated cost of radiation dose exposure and risks (cataract formation, optic neuropathy, and radiation-induced cancers). According to European Community and American guidelines, radiation dose reduction is a constant concern because of the fast growing use of CT, which leads to the principle of keeping dose exposure as low as reasonably achievable. In rhinology, dose-reduced protocols for conventional scans (i.e., sinusitis) do exist; however, only recently was radiation dose reduction assessed for CT-based navigation in FESS for clinically applied surface matching7 and preclinical experiments with rigid fixed fiducials.8 These studies showed that the differences in radiation absorption between tissue and air, as represented on the Hounsfield scale ( Fig. 31.1 ), are large enough to be resistant to diminishing signal-to-noise ratio and blurring of the CT image by dose reduction. Thus, stable surface matching and navigation with a significantly reduced CT dose are technically possible ( Figs. 31.2 and 31.3 ). A particularly good opportunity for reduction of iatrogenic induced radiation dose exposure is possible in bone window–based FESS if the need for high tissue contrast or contrast media application can be excluded (i.e., for the majority of surgical cases with uncomplicated CRS). Although exact surface matching is feasible, dose reduction leads to noisier pictures, which are subjectively perceived by each surgeon differently; thus, radiation dose cannot be reduced at all costs but should follow reduction limits, as depicted in Table 31.1.


Currently, DVT offers the most promising technological advances in the reduction of radiation exposure with approximate effective dose of 0.25 mSv as compared with standard chest radiograph imaging (0.02–0.05 mSv). Moreover, DVT hardware is small and easy to use, which facilitates repeated intraoperative imaging in high-risk surgery to track soft tissue shift, imprecise resection, or complication. However, semicircumferential volume scanning with a cone beam still requires several seconds and is thus prone to movement artifacts. As a low-dose technology, DVT is excellent in imaging bony contours or implants, yet it lacks adequate soft tissue contrast and gradients as addressed by the Hounsfield scale.
Tips and Tricks
Because DVT (also known as cone beam CT) can drastically reduce radiation exposure for diagnostic imaging, it can more easily be used for multiple image acquisitions. This is particularly attractive for cases requiring follow-up imaging.

Position-sensing and -tracking Systems
The spatial position1 of surgical instruments and patient anatomy is measured by tracking systems that use mechanical, optical, or electromagnetic sensing methods, explained later in this section. Technically, spatial positions can also be measured through the bending of optical fibers or the traveling time of ultrasound signals, but these techniques remain unable to fulfill requirements in terms of accuracy, hysteresis, and repeatability needed for surgical applications.

Mechanical or electromechanical position sensors typically consist of an arm containing multiple rigid links, each possessing one or more rotational degrees of freedom such as the Viewing Wand, as seen in Fig. 31.4. Angles between successive joints are measured using rotary analog potentiometers or digital encoders. This information, together with the geometric properties of the arm segments, is then used to calculate the spatial orientation and position of the arm′s tip (typically a surgical probe). The first systems of this type consisted of five arm elements capable of measuring three positional and two rotational degrees of freedom of the attached probe. Although such systems were common at the beginning of IGS development, their awkwardness has now rendered them obsolete in today′s surgical navigation systems. However, extremely accurate examples of these measurement systems are still common in high-precision industrial applications.
Optical tracking systems are based on the detection of known geometric patterns using a set of optical cameras (typically two or three) arranged in a known geometric configuration. The 3D spatial position of a pattern can then be calculated from the planar positions in the several camera images using stereographic algorithms. Typical geometric patterns consist of several spherical or planar markers that can be stably and reproducibly detected by camera systems. The arrangement of such markers in a rigid (and defined) geometric manner and their capability to be tracked in dynamic situations leads to their name: rigid body, dynamic reference frame, or tracker. Passive tracking systems may use colored markers (video-based devices) or retroreflective/total-reflective spheres, whereas active tracking devices utilize light-emitting diodes (LEDs).
Typical optical tracking systems used in today′s surgical suites provide accuracies < 1 mm with a corresponding workspace of up to several cubed millimeters (Certus, Northern Digital Inc. [NDI], Waterloo, Ontario, Canada). Conversely, specialized systems can achieve much higher accuracies (µm) at the expense of workspace size (a few cubed centimeters).9 Thus, when developing novel IGS applications, the trade-off between required workspace and resolution must be weighed against factors associated with the physical size of the optical sensor and the line-of-sight problem. For example, the Vicra optical metrology camera from NDI ( Fig. 31.5a ) has a working volume of ~0.125 m3 and a root mean square (RMS) accuracy of 0.25 mm. In comparison, our experience with a new high-precision camera, the Cambar B1 (Axios Systems, Munich, Germany, Fig. 31.5b ), indicates that this measurement system has an RMS that is ~10 times smaller, although the workspace is only 0.6% of that of the Vicra system.


Electromagnetic tracking systems are based on analysis of electromagnetic field strength in perpendicular spatial orientations. Electromagnetic fields are generated by geometrically arranged coils within a generator unit. Spatial variation of the effective field strength is detected using small coils arranged in different spatial directions within a tracker. The alternating electromagnetic field (generator) induces corresponding voltages in the coils of the tracker. Analysis of the voltage patterns induced by differently aligned electromagnetic fields enables the determination of a position and an orientation of the tracker ( Fig. 31.6 ). One drawback of this measurement technology is the fact that electromagnetic fields are potentially susceptible to distortions caused by ferromagnetic metallic objects (operating room [OR] equipment, information technology [IT] equipment). The major advantage of electromagnetic tracking is that it is not dependent on direct line of sight between its hardware components and, therefore, can easily be integrated, even in cluttered operating theaters.
Note
Although stereo cameras offer the highest levels of tracking accuracy, the user must be aware of line-of-sight problems. A minimum of three tracking points (each tracking reference) must be visible by the camera during navigation.

Registration of Image Data with Patient Anatomy
Any IGS must spatially align different sets of information with each other using a so-called registration process. Image registration techniques vary in scope ( Fig. 31.7 ), and different approaches have evolved for dedicated clinical applications. Maintz and Viergever10 provided an extensive overview of available registration techniques. Typically, registration in the field of ENT surgery is achieved by aligning one or more image modalities (mostly 3D CT, MRI, or DVT image datasets) with the patient′s anatomy (see Video 40, Registering and Testing the Brainlab Navigation System ).
Image data registration begins with the identification of landmarks or fiducial markers, which are identified and highlighted in the image dataset through user identification of singular landmark points (i.e., edges of the front teeth, frontozygomatic suture, and center of fiducials) or (sub) surfaces of anatomical regions (i.e., the skin of the face). Corresponding anatomical landmarks are then identified on the patient, and their spatial positions are recorded preoperatively. Anatomical landmark acquisition is conducted by digitizing single points with a tracked instrument or through scanning of surfaces (i.e., using a laser scanner). Following landmark digitization, a rigid registration transformation is then calculated based on the spatial correlation of the landmark points (i.e., points are assumed to map one to one without any deformation). Following successful registration, the physical location of tracked instruments can be visualized relative to patient anatomy in a virtual scene.
Naturally, it is important to know the precision of the registration process to appreciate the uncertainty of the navigated tooltip position. Fiducial registration error (FRE) is a typical quantity (calculated from the RMS distance between corresponding fiducial landmarks) used to depict the approximate registration uncertainty. Although this value can directly be calculated, a more critical and direct registration error metric is the target registration error (TRE). This value provides rather realistic information on the actual surgery. However, TRE cannot directly be calculated, and only correlations between FRE and TRE have been identified in the past.11 Thus, available IGSs typically refer to FRE and require the surgeon to manually confirm the available individual accuracy after registration and prior to the actual instrument guidance phase.

Caution
FRE measurements are only an estimate of the accuracy of the tracked tool. Users should be aware that errors can be much larger than the reported value, and it is recommended that specific anatomical landmarks are used to verify the effective tracking accuracy.
Clinical Applications of Computer-aided Surgery
High-Precision Instrument Guidance in the Ear
Reduction of comorbidity and cost has recently extended implementation of minimally invasive surgical techniques into otorhinolaryngology (ORL).12 However, minimally invasive computer-aided microsurgery (CAMS) interventions require an unprecedented level of overall system accuracy. For example, inserting a cochlear implant in a single drill pass requires the definition of a drill trajectory through the facial recess to access the middle ear. This trajectory (~1–3.5 mm in width) is bounded posteriorly by the facial nerve and anteriorly by the chorda tympani. To successfully avoid these structures, an overall instrument guidance accuracy of 0.2 to 0.3 mm is required (see Table 31.2 for an error analysis). Bell et al13 presented a system capable of achieving an accuracy level of one order of magnitude below that of current CAS approaches ( Fig. 31.8 ).
The approach is based on using (1) DVT imaging with a maximum resolution of 0.15 × 0.15 × 0.15 mm3 and an effective radiation dose of 0.2 mSv; (2) a semiautomatic fiducial marker selection to optimize patient-to-image registration; (3) high-precision optical tracking with an RMS error < 0.02 mm in a tracking volume of 200 × 200 × 150 mm3; and (4) the development and application of a surgical robotic device to enhance the surgeon′s drilling capabilities and to facilitate a precise placement of implant cavities.
Augmented Reality
Image-guided surgery systems that deploy augmented reality technology allow surgeons to view pathologies (e.g., tumors) and vital anatomical structures in a more immersive environment. Especially applicable are anatomical structures hidden or beneath visible surfaces that can be visualized directly in the surgeon′s view. Among others, recent work has been published primarily in the field of visually augmented laparoscopy and microscopy.
Modern surgical microscopy systems allow for the augmentation of the original microscopic view with additional stereotactic guidance information or individual patient medical image data. To display a correct overlay image, changes in patient position must be determined. The use of external tracking systems has enabled tracking of a calibrated 3D object whose geometry in 3D space is known.14 The system can be used to overlay a segmented image (i.e., a tumor) directly in the surgeon′s microscopic view ( Fig. 31.9 ).


Another but similar approach is the application of endoscopic systems for the visualization of additional image data with respect to the spatial relationship between the patient and the optical instrument. The first of such systems were described for general image augmentation in the late 1990s.15 Today laparoscopic augmentation is now available as part of modern IGS suites. Actual research focuses on a dedicated visualization of medical image data such as anatomical landmarks16 that have been identified by the surgeon prior to surgery. The landmarks are displayed as circular dots with a predefined color, together with their name and their distance to the endoscope′s tip. The surgeon profits from better understanding and orientation in the surgical site. Additionally, the appearance (size, shape, and color) of the visualized landmarks can be programmed to vary depending on the proximity of an instrument or endoscope to it and hence can function as an alert.
Virtual Reality and Simulation
Ever since the availability of sufficient computing power and 3D image quality, the 3D visualization of organs or body cavities with a lumen, including the gastrointestinal tract, trachea and bronchi, vessels, urinary tract, inner and middle ear, and paranasal sinuses, has been of great interest. Virtual models of the nasal cavity, nasopharynx, or the paranasal sinuses and visualizations thereof have been demonstrated.17 Ongoing research tries to improve available image quality using such techniques as volume rendering, ray casting, and increasing the level of visible details by applying material properties to the visualized parts. Furthermore, this technology has been applied to individual surgery planning as well as educational purposes. Figure 31.10 is a typical example of the use of rendering and material properties to create a realistic visualization of the sinus sphenoidalis.

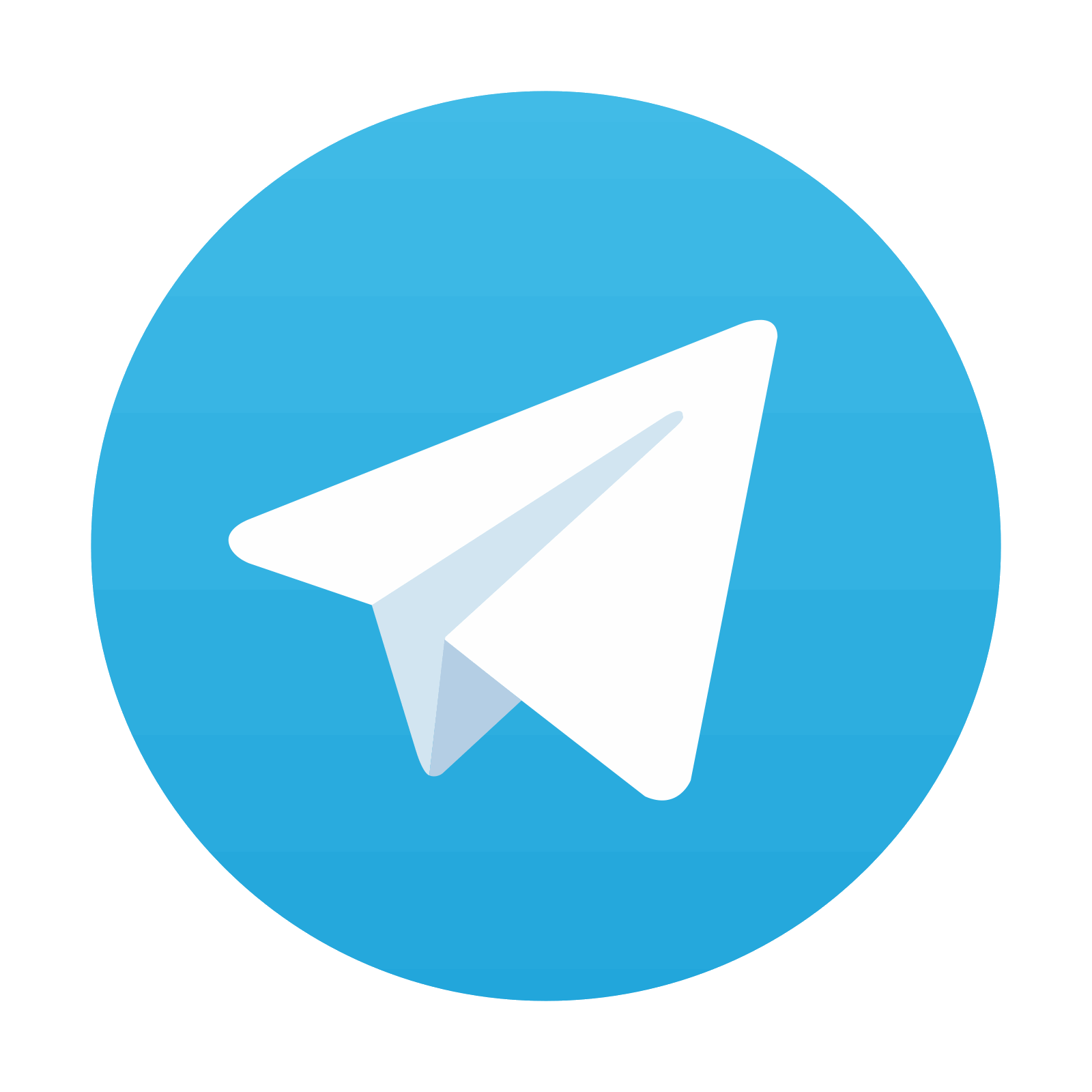
Stay updated, free articles. Join our Telegram channel
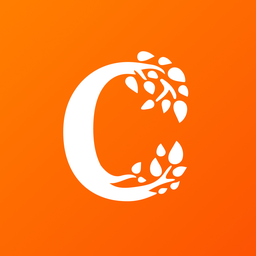
Full access? Get Clinical Tree
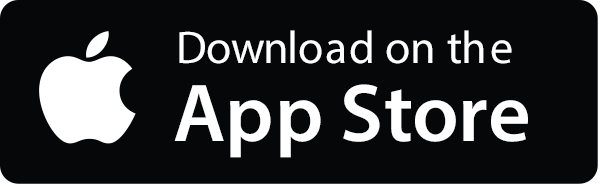
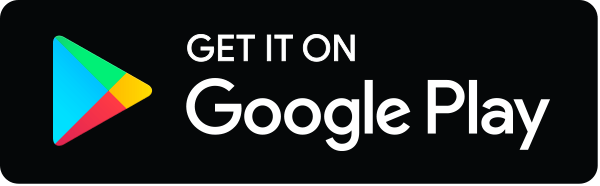
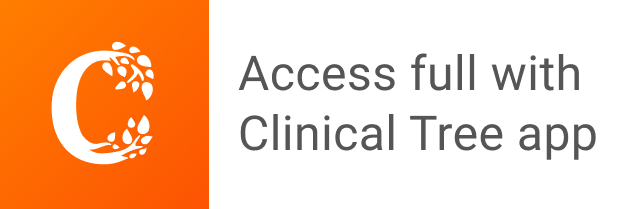