Introduction
Somatosensory innervation of the eye is provided by the peripheral axons of a small number of primary sensory neurons located in the trigeminal ganglion (TG). Most sensory axons enter the eyeball through the long and short ciliary nerves and reach all ocular tissues with the exception of the lens and the retina. Ocular innervation is particularly rich in the cornea, although all tissues of the anterior segment of the eye have an abundant sensory supply. Ocular sensory nerve fibers are functionally heterogeneous and include low mechanoreceptor, mechano- and polymodal nociceptor, and cold thermoreceptor types of sensory units responding to a variety of physical and chemical stimuli. Impulse activity originated at peripheral sensory nerve fibers innervating the eye reaches second-order neurons located at the trigeminal nucleus caudalis in the lower brainstem, from where sensory information travels to relay stations in the contralateral posterior thalamic nucleus and finally reaches the brain cortex.
Pain is the main sensation elicited by stimulation of ocular sensory nerves, although tactile sensations are evoked at the conjunctiva and feelings of cold are also evoked by mild cooling of the ocular surface. After repeated noxious stimulation or tissue injury, ocular polymodal nociceptors become sensitized and cause sustained pain and hyperalgesia. They also contribute to local inflammation through the antidromic release of peptide neurotransmitters stored in their peripheral endings (the “neurogenic inflammation”). Intact sensory nerve endings, particularly in the corneal epithelium, are subjected to a continuous remodeling. Ocular sensory nerves damaged by accidental or surgical injury or as a consequence of pathologic processes may also experience long-lasting changes of their excitability, giving rise to ocular dysesthesia and neuropathic pain referred to the eye.
Sensations originated at the ocular surface can be explored by esthesiometry. The cornea has an overall higher sensitivity than the conjunctiva. Sensitivity of the ocular surface is reduced by age and by a variety of pathologic processes that affect sensory innervation, such as herpetic keratitis, some types of corneal trauma and postinfectious conditions, certain hereditary corneal dystrophies, and anterior segment or vitreoretinal surgery. Although the principal eye diseases leading to impaired vision—such as retinal pathologies, chronic open-angle glaucoma, or cataract—course without pain, pain is a cardinal symptom of inflammatory and traumatic disturbances of the anterior segment of the eye. These include postsurgical conditions that may lead to ocular neuropathic pain, that is, pain caused by injury or disease of the ocular somatosensory system, either in the peripheral nerves or the central structures involved in ocular sensitivity. Dry eye, a multi-etiological condition that leads to the alteration of the ocular surface and its innervation, is the most common cause of ocular discomfort and pain.
Anatomy of ocular sensory nerves
Origin of the ocular sensory nerves
Trigeminal ganglion neurons
The sensory innervation of the eye is provided by primary sensory neurons (most of them of small or medium size), clustered in the ophthalmic (medial) region of the ipsilateral TG. Ocular sensory neurons represent about 1.5% of the total number of neurons of the TG, most of them devoted to the cornea. Trigeminal neurons are pseudounipolar and their axons divide into a peripheral branch that projects to the peripheral target tissues and a central myelinated branch that enters the brainstem to reach the trigeminal sensory complex. Based on the size and the presence (or absence) of a myelin sheath in the peripheral axons, corneal TG neurons can be classified as myelinated and unmyelinated (20% and 80% in the mouse, respectively). The physiologic significance of myelin content is reflected in the conduction velocity of the peripheral axons (see further).
Most TG neurons innervating the eyeball contain several neuropeptides typical of primary sensory neurons, including calcitonin gene–related peptide (CGRP, present in about 50% of corneal neurons), the tachykinin substance P (present in about 20%), cholecystokinin, somatostatin, opioid peptides, pituitary adenylate cyclase–activating peptide (PACAP), vasoactive intestinal peptide (VIP), galanin, and neuropeptide Y (NPY), as well as neuronal nitric oxide synthase (nNOS).
The ophthalmic nerve and its branches
The peripheral axonal branch of most ocular sensory neurons in humans exits the TG running with the ophthalmic division of trigeminal nerve (fifth cranial nerve), which traverses the superior orbital fissure and then branches into the nasociliary, the frontal, and the lacrimal nerves ( Fig. 16.1 ). The nasociliary nerve gives several sensory branches: (1) two long ciliary nerves that reach the eyeball and pierce the sclera, constituting the major somatosensory output of the eye; (2) the infratrochlear nerve that innervates the medial aspect of lids, nose, and lacrimal sac; (3) the external nasal nerve that innervates the skin of the nose, the ala nasi and the nasal vestibule; and (4) a communicating branch to the ciliary ganglion. The ciliary ganglion is a parasympathetic ganglion located within the orbit that sends 5 to 10 short ciliary nerves carrying parasympathetic postganglionic axons, postganglionic sympathetic axons originating at the superior cervical ganglion, and the trigeminal sensory nerve axons arriving through the communicating branch of the nasociliary nerve. These mixed, short ciliary nerves enter the eyeball around the optic nerve. The second branch of the ophthalmic nerve is the frontal nerve, which bifurcates into the supraorbital nerve to innervate the upper eyelid and the frontal sinus, and the supratrochlear nerve that provides innervation to the forehead and the upper eyelid. Finally, the third branch of the ophthalmic nerve is the lacrimal nerve, which also incorporates postganglionic parasympathetic nerve fibers from the pterygopalatine ganglion and innervates the lacrimal gland and some areas of the conjunctiva and the skin of the upper lid.

A minor part of the sensory axons arising from the eye is conveyed together with the innervation of the conjunctiva and the lower eyelid skin, by the infraorbital nerve, a branch of the maxillary nerve, which in turn constitutes the second major branch of the TG ( Fig. 16.1 ).
Trigeminal sensory axons end in the epithelia, connective tissue, and blood vessels of the lids, orbit, extraocular muscles, ciliary body, choroid, iris, scleral spur, sclera, cornea, and conjunctiva—the retina and lens being the only ocular tissues not receiving direct trigeminal sensory innervation.
Distribution of sensory nerve fibers within the eye
After penetrating the sclera around the optic nerve at the posterior eye pole, long and short ciliary nerves containing a mixture of sensory, sympathetic, and parasympathetic axons form a ring around the optic nerve, keeping the lamina cribrosa devoid of innervation. From there, ciliary nerve fascicles travel anteriorly toward the cornea within the suprachoroidal space, giving a few collaterals that provide the sparse innervation of the posterior part of the eye. Along their trajectory to the front of the eye, these nerve fiber bundles undergo repetitive branching. Some of the resulting nerve fibers innervate the sclera itself, especially the episcleral portion; others leave the sclera to enter the choroid, while most nerve filaments continue to innervate the ciliary body, the iris, and the cornea. Most of them finally form a series of ring meshworks of nerve fibers at the limbus—the so-called limbal or pericorneal plexuses.
Nerve filaments entering the choroid are composed of sensory, sympathetic, and parasympathetic axons, and branch extensively forming a dense network in which individual parasympathetic ganglion cells are also found.
Limbal plexus nerves supply the sensory and autonomic innervation of the limbal vessels, the trabecular meshwork, and the scleral spur. Nerve bundles also form a circumferential plexus at the root of the iris that gives origin to the iridal innervation. Finally, a variable number of nerve trunks arising from the limbal plexus enter the corneal stroma (corneal stromal nerves). The cornea receives a significant portion of the ocular innervation, presenting a nerve density that is estimated to be 300–400 times higher than that of the human fingers or the teeth.
The majority of the nerves directed to the eye are composed of thin, unmyelinated nerve fibers often incompletely surrounded by Remak (nonmyelinating) Schwann cells, while less than 30% present a myelin sheath provided by myelinating Schwann cells. Ocular nerves branch extensively, and most of them terminate as free nerve endings showing small enlargements (varicosities) along their terminal course. In addition, some encapsulated nerve endings similar to Krause and Meissner bodies have been observed in the choroid, in particular near the ciliary body and the iris, and in the episclera and chamber angle but not in the cornea.
Architecture of corneal sensory nerves
The corneal innervation is anatomically organized in four levels from the penetrating stromal nerve trunks up to the intraepithelial nerve terminals ( Fig. 16.2A ).

Corneal stromal nerves
The anatomy of nerve trunks radially entering the corneal stroma is quite similar among mammals, varying only in their number (6–8 in rat, 15–40 in cat or dog, about 60 in human). Stromal nerves branch immediately after entering the cornea and run within the stroma as ribbon-like filaments enclosed by a basal lamina and Schwann cells. Stromal nerve trunks are composed of both unmyelinated and thinly myelinated (Aδ) axons ( Fig. 16.2B ), although myelinated axons (about 20–30% of the nerve fibers) lose their myelin sheath within a millimeter after penetrating the stroma. Similarly, nerve fascicles also lose the intrinsic blood vessels once in the stroma, which contributes to the transparency of the cornea. The branches of the stromal nerve arborization anastomose extensively, forming the anterior stromal nerve plexus, a dense and complex network of intersecting small- and medium-sized nerve bundles and individual axons with no preferred orientation laying in the anterior corneal stroma, at different depths depending on the species but being the densest in the anterior third of the stroma ( Fig. 16.3A,B ). In contrast, the posterior half of the human stroma and the corneal endothelium are devoid of sensory nerve fibers.

In addition to the stromal nerve innervation, a few small nerve fascicles originating at the limbal plexus and at the conjunctiva superficially enter the peripheral cornea to innervate the perilimbal and peripheral corneal zones.
Subepithelial nerve plexus
In humans and higher mammals, the most superficial layer of the anterior stromal nerve plexus, located in a narrow strip of stroma immediately beneath Bowman’s layer, is especially dense and is referred to as the corneal subepithelial nerve plexus, its nerve density generally higher in the peripheral than in the central cornea. Two anatomically distinct types of nerve bundles are distinguished in the subepithelial plexus. One forms a highly anastomotic meshwork made of single axons and thin nerve fascicles located immediately beneath Bowman’s layer without penetrating it, toward the corneal epithelium. The second type consists of about 400–500 medium-sized, curvilinear bundles that penetrate Bowman’s layer and the epithelium basal membrane, mainly in the peripheral and intermediate cornea. These bundles shed the Schwann cell coating, bend at a 90-degree angle, and divide, each into 2 to 20 thinner nerve fascicles that continue into the corneal epithelium as the sub-basal nerve plexus ( Fig. 16.2A,B ; Fig. 16.3A,C,D ). A relatively low number of stromal nerves penetrate Bowman’s layer in the central cornea, which receives most of its innervation from long sub-basal nerves that enter the peripheral corneal epithelium directly from the limbal plexus. Remak Schwann cells cover nerve axons within the stroma until the nerve bundles traverse the epithelium basal membrane. Once in the epithelium, nerve axons are no longer covered by glial cells ( Fig. 16.2.B ).
Despite their considerable branching, most stromal and subepithelial nerve bundles pass uninterrupted through the stroma to reach the corneal epithelium and apparently do not provide functional innervation to stromal tissue. However, a small proportion of corneal nerve fibers appear to travel downward to terminate in the stroma as expanded structures resembling free nerve endings or in close relation with stromal keratocytes and resident immune cells.
Sub-basal nerve plexus
The sub-basal nerve plexus in humans is formed by 5000 to 7000 nerve fascicles inside an area of about 90 mm 2 . A sub-basal nerve bundle gives several side branches, each containing three to seven individual axons. Thus, the total number of axons in the sub-basal plexus is estimated to vary between 20,000 and 44,000.
The sub-basal nerve axons may travel up to 6 mm between the basal epithelial cells and their basal lamina, roughly parallel to one another. The arrangement of a stromal nerve bundle branching into multiple, parallel daughter nerve fascicles constitutes a unique neuroanatomical structure characteristic of the cornea and is termed an epithelial leash. Epithelial leashes are constituted by up to 40 individual unmyelinated straight and beaded nerve fibers of variable diameter (0.05–2.5 microns). Nerve fibers in adjacent leashes interconnect repeatedly such that they are no longer recognizable as individual leashes, forming, finally, a relatively homogeneous nerve plexus. In vivo confocal microscopy (IVCM) has shown that the sub-basal nerve plexus often forms a whorl-like spiral pattern of nerve fibers, the center of which is called the “vortex” ( Fig. 16.3A ). In humans it is located 2 to 3 millimeters inferior and nasal to the corneal apex. Many mammal species including mouse, rat, guineapig, cat, dog, and macaque also present this centripetal spiral whorl-like nerve pattern. Most species have only one spiral whereas multiple spirals are present in the mouse cornea ( Fig. 16.3A ). The mechanisms that govern the formation and maintenance of this spiral pattern remain unknown. Basal corneal epithelial cells and sub-basal nerves appear to migrate centripetally in tandem during development and in the adult. Thus it is possible that basal epithelial cells derived from limbal stem cells migrate centripetally in a whorl-like fashion toward the corneal apex in response to a chemotropic guidance, electromagnetic cues, population pressures, and/or preferential desquamation of epithelial cells in the central cornea. The sub-basal nerves that occupy the narrow intercellular spaces between these migrating cells would be pulled along their trajectory. An alternate possibility is that sub-basal nerves would develop first their whorl-like orientation, providing in turn a structural scaffolding that directs epithelial cell migration, or it may be that both nerves and epithelial cells follow the same cues, either when moving at the same time or at different times during development.
Intraepithelial nerve terminals
From the sub-basal nerves running horizontally through the basal epithelium, single fibers split off and turn 90 degrees vertically as a profusion of thin, short, and beaded terminal axons ascending between the epithelial cells, often with a modest amount of additional branching, up to the more superficial layers of the corneal epithelium ( Fig. 16.3C–E ). Depending on their branching pattern, intraepithelial nerve terminals are described as simple, ramifying, or complex ( Fig. 16.3C–E ). Some nerve terminals do not branch after leaving the sub-basal nerves and end as free nerve endings within the outermost cell layer of the corneal epithelium, being classified as simple intraepithelial nerve terminals. Ramifying nerve terminals branch between the wing and squamous cell layers of the epithelium into three to four parallel nerve fibers traveling for up to 100 μm. Complex nerve terminals branch profusely within the wing cell layer of the epithelium, forming clusters of fibers that end both between the wing and squamous cell layers. All three types of intraepithelial fibers end as free nerve endings, appearing as prominent, bulbous terminal expansions that appear morphologically homogeneous when visualized using optical or electron microscopy, although immunocytochemical staining reveals differences in the expression of neuropeptides and other neurotransmitters, suggesting a functional heterogeneity. At the ultrastructural level, corneal nerve endings contain abundant small clear vesicles, perhaps filled with excitatory amino acids, and large, dense-cored vesicles containing neuropeptides such as calcitonin gene–related peptide (CGRP), substance P, and other peptides. They also contain mitochondria, glycogen particles, neurotubules, and neurofilaments. The nerve terminals are located throughout all layers of the corneal epithelium, extending up to a few microns of the corneal surface. Occasionally, epithelial cell membranes facing the nerve terminals show invaginations that may eventually completely surround the nerve ending. This intimate relationship raises the possibility of bidirectional exchange of diffusible substances between both structures. Epithelial cells may also function as substitute glial cells for intraepithelial axons, phagocytizing distal axon fragments within hours after corneal nerve lesion. The intimate contacts also allow nerve endings to detect changes in epithelial cell shape or volume, such as those produced by ocular surface desiccation or swelling, thus contributing to corneal sensitivity.
The innervation density of the corneal epithelium is probably the highest of any surface epithelium. Although the actual number of corneal nerve endings remains a matter of speculation, considering that each sub-basal nerve fiber gives at least 10 to 20 intraepithelial nerve terminals, it is reasonable to speculate that the human central cornea contains approximately 3500 to 7000 nerve terminals/mm 2 . This rich innervation provides the cornea with a highly sensitive detection system, and it has been hypothesized that injury of a single epithelial cell may be sufficient to trigger pain perception. Nerve terminal density, and thus corneal sensitivity, is higher in the central cornea and decreases progressively when moving toward the periphery. Similarly, corneal sensitivity and nerve density decrease progressively as a function of age and in several ocular pathologies, including systemic metabolic diseases such as diabetes, and ocular viral, parasitic, and bacterial infections ( Table 16.1 ).
Reduced sensitivity | |
---|---|
Genetic diseases |
|
Other systemic diseases |
|
Eye surgery |
|
Infections |
|
Drugs |
|
Other conditions |
|
Enhanced sensitivity or irritation sensations | |
Diseases of the ocular surface |
|
Acute or chronic inflammation of the ocular surface |
|
An individual stromal axon entering at the corneoscleral limbus undergoes repetitive branching and eventually travels across as much as three-quarters of the cornea before terminating. As a result, individual receptive fields of corneal sensory fibers range in size from less than 1 mm 2 to as much as 50 mm 2 and may cover up to 25% of the corneal surface. The extensive branching also explains the significant overlapping of receptive fields found in electrophysiological studies of single corneal nerve fibers.
Morphology of conjunctiva and lid margin sensory innervation
Compared with the cornea, little is known about the sensory innervation of the conjunctiva and eyelid margins. Sensory innervation of the conjunctiva and lid margin is provided by the supratrochlear, infratrochlear, supraorbital, and lacrimal nerves (all of them branches of the ophthalmic nerve), and the infraorbital nerve (branch of the maxillary nerve). Nerve bundles innervating the conjunctiva are thin myelinated and unmyelinated axons containing CGRP and/or substance P and terminating as free nerve endings located around the stromal blood and lymph vessels. Some nerve endings locate in the conjunctival epithelium or around the acini of meibomian glands. Myelinated axons terminating at the limbal conjunctiva as structures resembling Krause nerve endings have been described in the human eye. They are distributed in all the bulbar conjunctiva but are more abundant around the limbus.
The lid margin is innervated by free nerve endings, mostly located within the epithelial cells at the mucocutaneous junction, as well as by abundant structures similar to the Merkel disc endings and Meissner corpuscles described in the skin. Similarly to the hairy skin, the lid margin is also innervated by myelinated axons ending as lanceolate terminals associated with clusters of Merkel cells, encapsulated Ruffini endings and/or with the base of eyelashes. These are specialized structures associated to low-threshold mechanoreceptors that may provide extreme mechanical sensitivity of the eyelid margin, where protective reflex blink responses are evoked even by mechanical stimuli of very low intensity.
Central sensory pathways
Trigeminal brainstem nuclear complex
Sensory information from the eye is carried by TG neurons to the ventral portion of the ipsilateral trigeminal brainstem nuclear complex (TBNC), to activate ocular sensory second-order neurons located at several levels of TBNC. Most second-order neurons receiving input from TG neurons innervating the cornea are found in the intermediate zone between interpolaris and caudalis subnuclei (Vi/Vc), in laminae I and II of the subnucleus caudalis/upper cervical spinal cord (Vc/C1), and in the adjacent bulbar lateral reticular formation ( Fig. 16.4 ). Additionally, few trigeminal neurons innervating ocular and periocular tissues project to the principal nucleus of the TBNC, and a very sparse number are confined to a few locations along the ventral border of the pars oralis and interpolaris of the spinal trigeminal nucleus.

A similar pattern has been described for the central connections of TG neurons innervating the eyelid and the lacrimal glands.
The different areas of the TBNC are interconnected through a dense fiber system. Its functional significance is not fully understood, but it seems to contribute to protective mechanisms integrated at the brainstem and driven by ocular sensory input, as is the case of reflex blinking.
Ocular representation at higher levels of the central nervous system
Most ocular second-order neurons project to different places in the central nervous system including brainstem regions as the superior salivatory/facial motor nucleus and the contralateral thalamus, although very little is known still about the central sensory pathway involved in ocular sensations. Neurons receiving corneal information have been identified in the most posterior and medial areas of the somatosensory thalamus, which in terms of somatotopy correspond to the representation of the trigeminal ophthalmic branch. These thalamic neurons project in turn to primary (SI) and secondary (SII) somatosensory cortical areas responsible for pain sensations and reactions. Nonvisual sensory input from the eye is represented in contralateral cortical SI areas 3b and 1 ( Fig. 16.4 ), located together with those that represent nose, ear, and scalp.
Some ocular second-order neurons at the Vi/Vc transition and the VcC1 region project to the parabrachial area, which is in turn connected with the insular, cingulate, and prefrontal cortex and the amygdala, hypothalamus, and periaqueductal gray matter. These projections provide the neural substrate for the affective components of sensations evoked by eye stimulation, as well as for the involvement of the autonomic nervous system in ocular pain.
Development, remodeling and regeneration of corneal innervation
Development of corneal nerves
The cornea lacks sensory innervation until the fifth gestational month, when corneal nerve endings appear. During development, sensory axons first form the nerve ring around the cornea (the limbal nerve plexus) and subsequently axons grow radially into the corneal tissue ( Fig. 16.5A ). The molecular signals controlling ocular nerve growth remain unknown, although several guidance molecules may regulate the process. Nerve growth factor (NGF; one of the neurotrophic factors released by corneal epithelial cells, keratocytes, and bone marrow–derived cells present in the cornea) influences the development and survival of corneal nerves. The human cornea also expresses other neurotrophins as brain-derived neurotrophic factor (BDNF), and neurotrophins-3 (NT-3) and 4 (NT-4). Regulators of cell movement and migration also contribute to guide the trigeminal axons during development. These regulators include Slits, netrins, ephrins, and semaphorins and their respective receptors: Roundabout (ROBO), UNC/DCC, Eph receptors, and neuropilins. Some members of the semaphorin family, in particular the so-called immune semaphorins, are expressed in corneal epithelium and regulate corneal nerve development by exerting different roles. Semaphorins promote axon outgrowth and also mediate, together with other nerve guidance molecules as Slit2, the initial repulsion of trigeminal sensory axons from the cornea, thus inducing the proper formation of the pericorneal nerve ring meshwork, and also contribute to the positioning of a subset of axonal projections in the choroid fissure that form a ventral plexus from which the iris innervation is later supplied. Upon completion of the pericorneal nerve ring, sensory nerves enter the cornea as bundles, at uniformly spaced sites around its entire circumference, and begin to extend radially inside the cornea, first innervating its periphery and then the entire stromal surface. The depth at which nerves enter the corneal stroma correlates with the area of the cornea that they will innervate, so that the deepest stromal nerves innervate almost the entire corneal surface, whereas the more superficial nerves that enter the stroma nearest to the epithelium mainly supply the periphery.

The uniform separation between corneal nerve bundles during embryogenesis seems to be due to the release of neurorepulsive factors from pioneering growth cones as they leave the limbal nerve ring and enter the corneal stroma, keeping nerve bundles growing relatively straight toward the center of the cornea as centripetal radii and separated one from another. Semaphorin 3 A, a secreted semaphorin expressed in the developing lens and cornea, and its receptors neuropilin11 and plexin-A4 present in trigeminal nerve sprouts, are key regulators of trigeminal axon branching in the cornea. While guiding nerve growth, semaphorins may also contribute to keeping the cornea avascular. Semaphorins repel angioblasts and vascular endothelial cells during development. The extension or radial innervation within the cornea is produced by bifurcation of nerve fascicles in successive, concentric zones, thus suggesting that the position and orientation of intracorneal nerves is determined by the intracorneal milieu and the migration of epithelial cells. However, sub-basal nerve arrangement in a swirl pattern seems to precede the centripetal epithelial cell migration to form the central cornea. Stromal composition and neurotrophic factors released from corneal epithelium also participate in the regulation of nerve density and orientation of corneal nerves during development.
Dynamic remodeling of adult corneal innervation
Corneal nerves are subjected to a remodeling in the adult cornea throughout life. Deep stromal nerve fiber bundles maintain a relatively constant position and configuration within the cornea, whereas the corneal sub-basal nerve plexus, and especially intraepithelial nerve terminals, experience an extensive rearrangement ( Fig. 16.5B ; Box 16.1 ). Time-lapse IVCM examination of living human eyes reveals that the human sub-basal corneal nerve plexus is a dynamic structure with a slow but continuous centripetal movement (5–15 microns/day) that changes the whorl-centered plexus in a 6-week period. Intraepithelial nerve endings are subjected to more prominent morphofunctional changes; their continuous remodeling follows the long-term sub-basal nerve reconfiguration. Glial cells do not appear to be involved, as there are no Schwann cells within the epithelium. The possibility exists that the continuous shedding of corneal epithelial cells strongly influences the rearrangement and dynamics of epithelial nerve terminals.
The continuous remodeling of corneal nerves may be disturbed by an altered corneal architecture or prolonged wound healing. Abnormal patterns in sub-basal nerve organization have been observed following corneal epithelial pathologies such as basement membrane dystrophy, recurrent corneal erosions, or dry eye.
Reorganization of differentiating corneal epithelial cells migrating up to the most superficial layers of the corneal epithelium induces changes in intraepithelial nerve ending architecture that occur continuously and can be appreciated from one day to the next. The molecular mechanisms responsible for this continuous rearrangement of corneal nerves are unknown. Adult corneal sensory nerves retain the ability to respond to semaphorins, which promote nerve sprouting both in transected and intact nerves, suggesting the semaphorins as drug targets to improve corneal nerve regeneration.
Changes of corneal sensory innervation with aging
In several mammalian species, corneal nerve terminal density decreases with age, and this may be the basis of the decreased corneal sensitivity and the altered tearing regulation reported in the elderly. Although corneal nerves may have uncompromised morphology and function throughout life, it is common for them to be subject to degenerative processes typical of aging. The availability of transgenic and knock-in mice allows the visualization of nerves in the transparent corneal tissue in living animals and ex vivo corneas, to study corneal nerve remodeling with age. The sub-basal plexus is fully developed in 3- to 4-month-old mice and starts disorganizing at 9 months. In old mice (18–24 months old), there is a reduction of sub-basal and intraepithelial nerve density, and an increased tortuosity and profound disorientation of sub-basal leashes that affect both the CGRP-positive axons (presumably nociceptors) and Transient receptor potential cation channel melastatin subfamily member 8 (TRPM8)-positive axons (presumably cold thermoreceptors). The proportion of straight and beaded nerve fibers of sub-basal leashes is also altered in aged animals, where most fibers are straight. Similarly, there are changes with age in the proportion of the different morphologic types of intraepithelial nerve endings, with simple intraepithelial nerve endings being abundant and complex nerve endings being very sparse in older animals.
Regeneration of injured corneal nerves
Like other peripheral nerves, adult corneal nerves retain the ability to regenerate following injury. After damage or transection, the morphology and functional properties of corneal nerves change substantially. Their morphologic and functional recovery depends on the extension, depth, and type of injury. After nerve transection, nerve processes distal to the site of lesion degenerate, whereas central stumps start to regenerate, finally producing a nerve pattern rather different from the original corneal nerve architecture. The regenerative process takes place in several stages ( Fig. 16.5C ). When corneal nerves are cut, the denervated area is first invaded by sprouts of adjacent, intact nerve fibers. Later, the central stump of injured axons starts to regenerate, forming microneuroma-like structures from which newly formed sprouts begin to develop, while the early branches from intact fibers begin to degenerate. Morphologic and functional changes in regenerating neurons may be due to the interruption of the uptake of signal molecules produced by corneal cells such as NGF. The absence of these molecules, usually centripetally transported along the parent axon to the neuron’s soma to regulate gene expression, causes morphologic and functional changes in regenerating neurons. Regeneration of corneal nerves after refractive vision correction surgeries or corneal transplant depends on the depth of ablation, considering whether or not it affects the stromal nerves. Whereas the fibers of the sub-basal plexus regenerate in months, especially from the sub-basal nerves external to the injury, the stromal nerves hardly regenerate and seem to stop at the edge of the injury. This is the reason why many corneal transplants completely lack stromal nerves, or these are limited to the peripheral area of the graft.
Corneal nerves are also negatively affected by many systemic and local diseases inducing neuropathies. Images suggestive of corneal nerve regeneration as the presence of neuroma-like structures and increased nerve tortuosity are common in herpes virus keratitis, diabetes, and dry eye. A highly prevalent systemic disease altering corneal nerve morphology and function is diabetes, in which corneal nerve density and sensitivity are reduced. In diabetic patients, the appearance of sub-basal nerves resembles the characteristics of intraepidermal small fiber neuropathy. Therefore, IVCM of corneal nerves could be used as a biomarker for the follow-up of diabetic neuropathy.
Functional characteristics of ocular sensory innervation
Most available data on the sensory neurons innervating the eye have been obtained by recording the electrical activity from the peripheral axons (innervating the cornea and the bulbar conjunctiva) or from the nerve terminals at the cornea of the primary sensory neurons located at the ophthalmic region of the TG. Knowledge is less abundant about the functional characteristics of the ocular trigeminal neurons, studied by recording the activity of TG neurons in situ or in tissue culture, or on the second-order ocular neurons located in the TBNC. Moving up the somatosensory pathway, knowledge about the somatosensory neurons that innervate the eye is even more scarce. These sensory neurons have been functionally classified depending on their response to different modalities of stimuli (mechanical, thermal, and chemical) ( Fig. 16.5 ).
Trigeminal ganglion neurons
Sensory neurons innervating the cornea
Mechanonociceptors
Around 15% to 20% of the nerve fibers innervating the cornea respond only to mechanical forces and are classified as mechanonociceptors. Their mechanical threshold is about 10 times lower than in the skin, possibly because corneal nerve terminals are more accessible to the stimulus, owing to its proximity to the surface of a nonkeratinized epithelium.
Corneal mechanonociceptors are medium-sized TG neurons whose peripheral axons are thinly myelinated and have conduction velocities in the Aδ range. They end as simple or sparsely ramified nerve terminals located in the uppermost cell layers of the corneal epithelium. The receptive fields are generally round and of medium size, covering about 10% of the corneal surface ( Fig. 16.6 ). The ability of mechanonociceptors to respond to mechanical stimulation is mediated by the mechanically sensitive ion channel Piezo2, expressed in the soma and peripheral nerve endings of corneal mechanonociceptor neurons. Their response to mechanical stimulation is phasic, firing only a few nerve impulses in response to both brief or sustained mechanical stimuli ( Fig. 16.7Ai ). Therefore, they probably serve mainly to signal the presence of noxious mechanical stimuli (touching of the corneal surface, foreign bodies, etc.), and are presumably responsible for the acute, sharp sensation of pain produced by sudden mechanical insults.
Polymodal nociceptors
More than 60% of the sensory neurons innervating the cornea and the bulbar conjunctiva are activated by stimuli of intensity within the noxious or near noxious range, including mechanical forces, heat, intense cold, exogenous chemical irritants, and a large variety of endogenous molecules released by injured corneal tissue and by resident and migrating immune cells participating in the inflammation response. Accordingly, they were named polymodal nociceptors ( Figs 16.6 & 16.7A,B ). Most of polymodal nociceptor neurons are small and medium-sized TG neurons that express the transducing channel TRPV1 and have unmyelinated (C) axons, while a small portion, depending on species, have thin, myelinated (Aδ) nerve fibers. Most corneal polymodal nociceptors and their axons in the cornea are peptidergic and express CGRP, and about two-thirds of them also contain substance P. They end as simple single-nerve terminals in the sub-basal plexus and the medium corneal epithelium layers, or as ramifying nerve terminals within the squamous cell layers. Their receptive fields are round or oval, usually large, often covering up to one-quarter or more of the cornea, and may extend several millimeters beyond the cornea onto the adjacent limbus and sclera ( Fig. 16.6 ). The large size and extensive overlapping of adjacent receptive fields of polymodal nociceptors, coupled with convergent mechanisms in the central nervous system, explain why stimuli of the corneal surface are poorly localized. Corneal polymodal nociceptors have tonic responses to the stimulation, with a continuous, irregular discharge of nerve impulses at a frequency roughly proportional to the magnitude of the stimulus, persisting as long as the stimulus is maintained and codifying its intensity and duration. Occasionally, the nerve impulse discharge overpasses the duration of the stimulus (postdischarge). Polymodal nociceptors respond to mechanical, chemical (local pH reduction), and heat (over 39–40°C) stimuli ( Fig. 16.7Aii,iii ). About half of them also develop a low-frequency response to corneal temperature reductions below 29°C. A large number of endogenous chemicals (inflammatory mediators) also activate polymodal nociceptors (see section Inflammation). Their ability to respond to different modalities of stimuli depends on their expression of different membrane transduction molecules ( Fig. 16.6C ). These include various members of the TRP (transient receptor potential) ion channels superfamily: (1) TRPV1 (transient receptor potential vanilloid type 1 ion channel), a heat-activated transducing channel that integrates the responses to injury and inflammation by its ability to be also activated by protons, several endogenous mediators, and external chemical irritants; (2) TRPA1, considered a chemoreceptor for environmental irritants and inflammation agents that is opened by exogenous irritant chemicals, natural toxins, bacterial LPS, pruritogenic agents, and noxious cold temperatures; and (3) TRPV2 (transient receptor potential vanilloid type 2 ion channel) and TRPV3 (transient receptor potential vanilloid type 3 ion channel), which are opened by high temperatures. Corneal polymodal neurons also express several members of the Acid-sensing ion channel (ASIC) family, which open in response to protons and endogenous mediators, and P2X channels opened by ATP, and presumably the mechanosensory channel Piezo2.



Cold thermoreceptors
About 10% to 15% of corneal nerve fibers discharge spontaneously at the resting temperature of the corneal surface (around 34°C). They increase their firing rate as soon as this temperature is decreased (which occurs, for example, during evaporation of the corneal surface tear film, or after application of cold solutions or air jets onto the cornea) and are transiently silenced upon warming. Corneal cold thermoreceptors have small receptive fields (around 1 mm diameter) located all over the corneal surface but more abundant in the peripheral cornea ( Fig. 16.6 ). Cold thermoreceptor axons end as richly branched complex nerve endings superficially located within the corneal epithelium, and are less frequently simple and ramifying nerve endings. Their sensitivity to cold is due to the expression of the cold-sensing channel TRPM8.
Depending on the level of expression of TRPM8 channels, two types of corneal cold thermoreceptors are present in the cornea of young and adult animals ( Fig. 16.6C ). About 60% of cold-sensitive neurons innervating the cornea and their peripheral nerve endings express abundant TRPM8 channels. They exhibit a high firing rate at the resting temperature of the ocular surface and are able to detect small corneal temperature decreases (≤0.5°C), which is the reason they are called high background activity–low threshold (HB-LT) cold thermoreceptors. The frequency of action potentials generated by HB-LT cold thermoreceptors encode the static temperature of the cornea, although they respond with a high frequency peak response to sudden temperature changes. HB-LT cold thermoreceptors also detect changes in osmolarity, increasing their firing rate when exposed to moderate hyperosmolarity (around 340–360 mOsm/L) and reducing their firing activity in the presence of hyposmolar solutions. The response to osmolarity changes also depends on TRPM8 channel. Under normal conditions, the activity of cold thermoreceptors is driven by the temperature reduction produced by tear film evaporation, as well as by the subsequent changes in tear film osmolarity.
Other cold thermoreceptor neurons show a weaker TRPM8 expression both in their somata and corneal nerve endings. Functionally, they correspond to a subpopulation of cold thermoreceptors with low firing rate at the background temperature of the cornea that require large temperature decreases to be activated (about –4ºC) and encode a wide range of corneal temperature decreases, being named low background activity–high threshold (LB-HT) cold thermoreceptors.
HB-LT cold thermoreceptors are recruited by small corneal temperature reductions, which are perceived as a sensation of freshness and cooling although with a variable irritative component. LB-HT cold thermoreceptors need more intense cooling of the cornea to be activated, being probably responsible for the unpleasant irritation and dryness sensations. In addition to evoking sensations, the information on corneal cooling and tear osmolarity changes provided to the central nervous system by cold thermoreceptors is used to regulate basal tearing and blinking rate.
Sensory neurons innervating the sclera, iris, ciliary body, conjunctiva, and lid margin
The functional properties of the sensory neurons innervating other parts of the eye globe, as well as the conjunctiva and lid margin, have not been studied in great detail yet. However, the same main functional classes of sensory afferents as in the cornea, i.e., mechanonociceptors, polymodal nociceptors, and cold receptors, have been identified in the sclera, iris and ciliary body, and the bulbar conjunctiva ( Fig. 16.6 ). Mechanosensitive and polymodal nociceptor fibers of the sclera, uvea, and cornea are readily activated by externally applied pressure but only transiently by sudden increases of intraocular pressure up to 100 mmHg, which explains the absence of pain in most forms of glaucoma. However, a combination of high intraocular pressure with inflammation, as occurs in congestive glaucoma, possibly causes nociceptor sensitization and a more sustained nociceptive inflow from ocular polymodal nociceptors to the brain, producing the intense pain characteristic for this disease. However, a small number of myelinated low-threshold mechanosensory nerve fibers responding to moderate changes of intraocular pressure and to mechanical stimulation have been functionally identified in the corneoscleral limbus and the lid margin. They may correspond to axons of the encapsulated nerve endings found in the chamber angle and scleral spur that may be associated with neural regulation of the intraocular pressure, and to the specialized structures associated with low-threshold mechanoreceptors present in the limbus and lid margin that are activated by airborne particles, as well as by blinking and contact lens wear.
Whereas almost all corneal polymodal nociceptor fibers are peptidergic, the conjunctiva contains both peptidergic and nonpeptidergic polymodal nociceptor fibers. Next-generation sequencing techniques have allowed the distinguishing in the conjunctiva of two main types of nonpeptidergic nociceptor fibers that are involved in mechanical pain and nonhistaminergic itch (expressing the oncogene-like MAS-related G protein–coupled receptor member D, MrgprD, innervating mostly the lid wiper), and in the conventional histaminergic itch (MAS-related G protein–coupled receptor member A3, MrgprA3, innervating the nasal and temporal conjunctiva). These pruritoceptors are not present in the cornea ( Figure 16.6 ).
Cold-sensitive nerve fibers with response properties similar to cold thermoreceptors present in the cornea are also found in the conjunctiva, especially in the bulbar conjunctiva, where their stimulation evokes pure cold sensations. Cold thermoreceptor nerve fibers also innervate the iris and the posterior sclera ( Fig, 16.6 ). Cold receptor endings in these locations are not exposed to environmental temperature changes but are close to blood vessels and may serve to detect choroidal and retinal blood flow changes, thus contributing to reflex blood flow regulation rather than to the production of conscious thermal sensations or basal tearing and blinking regulation.
Central pathways
Trigeminal brainstem nuclear complex
TG neurons innervating the eye and periocular tissues send the central projection of their axons to second-order neurons located at different levels of the TBNC. Most of them terminate at the lower part of the complex, the transition between the interpolaris and caudalis subnuclei (Vi/Vc), and the transition between the subnucleus caudalis and the cervical spinal cord (Vc/C1). Only a few TG neurons terminate at the upper part of TBNC, that is, the principal nucleus (Vp) and the subnucleus oralis (Vo). A modality-specific distribution of corneal neurons within the TBNC has been proposed: Vi/Vc neurons respond to all modalities of stimuli and are related to the control of blinking and tearing, whereas those within the superficial laminae of Vc/C1 area respond only to heat and chemical irritation, which suggests that the input to this region is restricted to TG polymodal nociceptor neurons. TG neurons innervating the eye lids and meibomian glands project over second-order neurons following a similar pattern.
Second-order neurons of the Vi/Vc transition have large receptive fields that cover almost the entire ocular surface. They encode mechanical, cold, heat, and chemical stimuli in the innocuous and noxious range, are sensible to changes in the level of moistness of the ocular surface, and become desensitized upon repeated stimulation. Some of them are also activated by intense light, probably those receiving projections of the small subset of photosensitive TG neurons containing melanopsin. These second-order ocular neurons project to the thalamus and to several brainstem regions as the superior salivatory or facial motor nucleus—depending on their peripheral input, and the accessory oculomotor nucleus ( Fig. 16.4A ). Their role in sensory-discriminative processing of ocular sensations seems minor, although Vi/Vc neurons participate in several trigeminal-evoked blink reflexes (initiated by mechanical stimulation, acidic or hyperosmolar solutions applied onto the ocular surface, bright light, etc.) in which afferent information from the ocular surface projects from the Vi/Vc to the superior salivatory nucleus and facial motor nucleus, responsible for the control of lacrimation and lid closing respectively. Likewise, a population of neurons specifically inhibited by wetting and excited by drying and cooling of the ocular surface may be involved in reflex tearing and fluid homeostasis of the ocular surface. We can speculate that these neurons receive at least part of their input originated at the peripheral cold fibers innervating eye surface tissues, whose sensory input is used to maintain basal lacrimation rate.
Second-order neurons of the Vc/C1 region also encode mechanical, cold, heat, and chemical stimuli in the noxious range, and bright light. Their receptive fields cover part of the ocular surface and the neighbor lid skin and are sensitized by repeated stimulation. Some of ocular Vc/C1 neurons also receive input from the dura mater, which suggests that they contribute to the development of headache. Vc/C1 ocular neurons project to the sensory thalamus, and also to the facial motor nucleus, the parabrachial nucleus, the amygdala, and the hypothalamus.
Little is known about neurons at Vp and Vo regions of the TBNC activated by stimulation of the periocular skin and the ocular surface, whose properties and contribution to ocular functions have not been yet established. Nevertheless, the development of aberrant “salt and pepper” ocular sensations in stroke patients with damaged lateral pons and medulla suggests a role for the Vp/Vo region in ocular sensory processing.
Thalamus and brain cortex
Going up along the ocular surface sensory pathway, studies are even scarcer, and no systematic mapping of the eye and periocular tissues has been yet performed. Neurons of the Vc/C1 region project to the posterior thalamus nucleus (see section Ocular representation at higher levels of the central nervous system), whose neurons project, in turn, to the amygdala and insular cortex rather than to the somatosensory cortex. So, it seems that very little information reaches the somatosensory cortex. In fact, early studies reported the absence of sensation evoked by electrical stimulation of the primary somatosensory cortex, an area that receives projections mainly from the somatosensory thalamus. It was assumed for decades that the surface of the eye was very poorly represented at the primary and secondary somatosensory cortex, until a recent case study using brain imaging pointed to the primary sensory cortex as a structure involved in ocular pain perception in humans ( Fig. 16.4B ). Supporting this, several studies in animals have reported the activation of primary somatosensory cortex neurons by mechanical stimulation of the cornea and periorbital tissues, and their differential response to noxious and non-noxious stimulation of the ocular surface.
However, there is evidence of the contribution to ocular sensory processing of various areas of the brain that are considered to be involved in the affective and behavioral aspects of sensory processing and, especially, in pain. This includes the anterior cingulate cortex, definite areas of the prefrontal cortex, and the insular cortex, whose electrical stimulation evokes pain and tingling sensations on the face and periocular area ( Fig. 16.4B ). This may explain our low capacity to evaluate with precision the sensory-discriminative aspects of the stimuli acting on the ocular surface, and why they are usually experienced as irritation or pain, with an intense and negative affective component and evoking nocifensive behaviors. The central processing of sensory information originating at the ocular surface evokes unique sensations, unmatched in other areas of the body, including those innervated by the TG. Whereas mechanical stimuli evoke clear tactile and cold sensations when applied to the skin, including the face skin, in the cornea they evoke sensations defined as gritty eyes, irritation, or pain.
In summary, sensory information initiated by the activation of sensory nerve endings at the eye surface and periocular tissues is processed by the central nervous system and leads to pain perception and affect, while evoking protective behavioral responses. First, the trigeminal primary sensory neurons detect the noxious stimulus acting over the ocular surface and send this information to TBNC neurons that, in turn, engage autonomic and motor brainstem circuits responsible for protective reflex responses (tearing, blinking). These brainstem-integrated reflexes reduce or avoid the exposure to the acting stimulus while the information is sent to upper levels of the central nervous system following two different pathways. In one of these pathways, ocular information is processed at the parabrachial area and then the hypothalamus and the amygdala, which in turn project to the insular, anterior cingulate, and prefrontal cortex, areas belonging to the brain circuits involved in the affective-motivational dimension of pain. Ocular information is processed in parallel by a second pathway where the sensory thalamus and the somatosensory cortex analyze the sensory-discriminative components. Altogether, brain processing will evaluate the sensory-discriminative and affective-motivational components of the multidimensional pain perception, leading to the ocular pain perception itself, to the activation of descending pathways of pain modulation, and to complex adaptative behaviors derived from recalling our previous experience and understanding the context (see section Sensations arising from the eye).
Trophic effects of ocular primary sensory neurons
As well as their role in signaling external or internal stimuli, ocular sensory neurons play an important role in the trophic maintenance of their innervated tissues, an effect that is particularly prominent in the cornea. Impairment of corneal sensory innervation causes a number of functional disturbances and the development of epithelial defects and recurrent ulcers (neurotrophic keratitis), which leads finally to loss of corneal transparency. Any condition that may damage corneal innervation (including cornea, lens and retina surgeries, infections, drug treatments, trauma, contact lens wear, genetic ocular and systemic diseases, and other systemic or ocular diseases) results in corneas with reduced sensitivity and altered healing capabilities, increased epithelial permeability, punctate staining following instillation of fluorescein or Rose-Bengal, poor healing of corneal ulcers, and, finally, reduced transparency. In patients with decreased corneal sensitivity, small epithelial defects expressive of an altered innervation may be seen in the cornea or the conjunctiva, usually in the areas not covered by the lids and, therefore, continuously exposed to the environment.
Many molecules may act as regulators that stimulate migration, proliferation, differentiation, and adhesion of corneal epithelial cells during wound healing. These include growth factors and cytokines (the epidermal growth factor family, basic fibroblast growth factors, transforming growth factors α and β, platelet-derived growth factor, insulin-like growth factor, keratinocyte growth factors, tumor necrosis factor-α, and several interleukins such as IL1, IL6, and IL10) that are present in the cornea and are activated as part of the wound healing process. This is also modulated by other effectors such as neuropeptides (CGRP, SP, cholecystokinin, etc.), ATP (released immediately after injury and acting through P2Y and P2X receptors), Toll-like receptor 4, protein kinases associated to Rho-GTPases, and the extracellular matrix components. Upon activation by the adequate stimuli, corneal sensory neurons generate action potentials that are conveyed to the central nervous system and also invade all axon branches of the neuron ( Fig. 16.5D ). This electrical depolarization of all the axon nerve endings releases neuropeptides locally, in particular SP and CGRP, that either by themselves or in combination with other growth factors present in the cornea and/or in corneal nerves (e.g., insulin-like growth factor, epidermal growth factor, PACAP, cholecystokinin, gastrin, NGF, etc.) appear to be important agents in maintaining corneal epithelial integrity and promoting wound healing in the normal cornea. Therefore, any situation that leads to a partial or total loss of the corneal innervation leads to a reduced activity of corneal nerves and subsequent trophic problems of the corneal tissue.
However, ocular tissues produce several growth factors necessary for the development, remodeling, and regeneration of corneal nerves. Growth and neurotrophic factors such as glial cell line–derived neurotrophic factors (GCNF), BDNF, opioid growth factor (OGF), ciliary neurotrophic factor (CNTF), pigment epithelium–derived factor (PEDF), vascular endothelial growth factor (VEGF), semaphorin A7, NGF, and NT-3 and NT-4 have been found in the cornea (produced by either the cellular components of the cornea or by its resident immune cells) and possibly participate in the maintenance of corneal innervation ( Box 16.2 ).
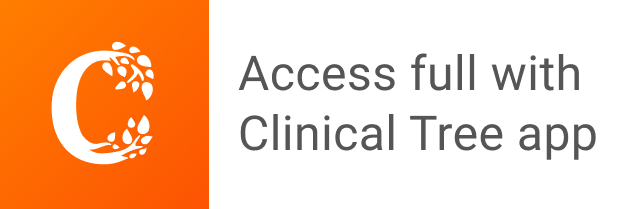