Introduction
The sensory innervation of the eye is provided by the peripheral axons of primary sensory neurons located in the trigeminal ganglion. The sensory nerves enter the eyeball mainly through the ciliary nerves and reach all ocular tissues with the exception of the lens and the retina. Ocular innervation is particularly rich in the cornea but all tissues of the anterior segment of the eye have an abundant sensory supply. Ocular sensory fibers are functionally heterogeneous and include mechanoreceptor, polymodal nociceptor, and cold receptor types of sensory units. Consequently, the nerves respond to a variety of physical and chemical stimuli. Impulse activity originated at peripheral sensory nerve fibers of the eye reaches second-order neurons located at the trigeminal nucleus caudalis in the lower brainstem. Sensory information travels subsequently to relay stations in the contralateral posterior thalamic nucleus and finally reaches the cortex.
Pain is the main sensation elicited by stimulation of ocular sensory nerves although tactile sensations are evoked at the conjunctiva and feelings of cold are also evoked by mild cooling of the corneal surface. After repeated noxious stimulation or tissue injury, ocular polymodal nociceptors become sensitized and cause sustained pain and hyperalgesia. They also contribute to local inflammation through the antidromic release of peptide transmitters stored in their peripheral endings (“neurogenic inflammation”). Intact sensory nerve endings, particularly in the corneal epithelium, are subjected to a continuous remodeling. Ocular sensory nerves damaged by accidental or surgical injury or following pathological processes, may also experience long-lasting changes in excitability, giving rise to ocular dysesthesia or neuropathic pain referred to the eye.
Sensations originated at the ocular surface can be explored by esthesiometry. The cornea has an overall higher sensitivity than the conjunctiva. Sensitivity of the ocular surface is reduced by age and by a variety of pathological processes that affect sensory innervation such as herpetic keratitis, some types of corneal trauma and post-infectious conditions, certain hereditary corneal dystrophies and anterior segment or vitreo-retinal surgery, especially scleral buckling operations. Although the principal eye diseases leading to impaired vision – such as retinal pathologies, chronic open-angle glaucoma or cataract – course without pain, pain is a cardinal symptom of inflammatory and traumatic disturbances of the anterior segment of the eye. Pain is also evoked by various ocular diseases affecting other structures within the globe or orbital tissues, and may become an undesired consequence of ocular surgery. Dry eye, a multietiological condition that leads to alteration of the ocular surface epithelium, is the most common cause of superficial ocular discomfort and pain. Management of ocular pain includes the use of topical anesthetics, cycloplegic agents and anti-inflammatory drugs or ocular patching to reduce peripheral nociceptive input, and systemic analgesics to act on nociceptive pathways at the central nervous system.
1
Anatomy of ocular sensory nerves
1.1
Origin of the ocular sensory nerves
Trigeminal ganglion neurons
The sensory innervation of the eye is provided by primary sensory neurons mainly of small or medium size, clustered in the ophthalmic (medial) region of the ipsilateral trigeminal ganglion (TG). Most ocular sensory neurons are devoted to the cornea and represent about 1.5 percent of the total number of neurons of the TG. The axons of the pseudounipolar trigeminal neurons divide into a peripheral branch that projects to the peripheral target tissues and a central branch that enters the brainstem to reach the trigeminal sensory complex. Based on the size and the presence of a myelin sheath in the peripheral axons, corneal trigeminal neurons can be classified as myelinated (20 percent in the mouse) and unmyelinated (80 percent in the mouse). Myelin content is reflected in the conduction velocity of the peripheral axons (see below).
TG neurons innervating the eyeball contain several neuropeptides, including CGRP (calcitonin gene-related peptide, present in about 50 percent of corneal neurons), the tachykinin substance P (present in about 20 percent), cholecystokinin, somatostatin, opioid peptides, pituitary adenylate cyclase activating peptide (PACAP), vasoactive intestinal peptide (VIP), galanin and neuropeptide Y (NPY), as well as neuronal nitric oxide synthase (nNOS).
The ophthalmic nerve and its branches
The peripheral axonal branch of most ocular sensory neurons in humans exits the TG running with the ophthalmic division of the ganglion, which traverses the superior orbital fissure and then branches into the nasociliary, the frontal and the lachrymal nerves. The nasociliary nerve gives several sensory branches: (1) two long ciliary nerves that reach the eyeball and pierce the sclera, constituting the major sensory output of the eye; (2) the infratrochlear nerve that innervates the medial aspect of lids, nose, and lachrymal sac; (3) the external nasal nerves; (4) a communicating branch to the ciliary ganglion. The ciliary ganglion is a parasympathetic ganglion located within the orbit that sends 5–10 short ciliary nerves carrying parasympathetic postganglionic fibers, postganglionic sympathetic axons originating at the superior cervical ganglion, and the trigeminal sensory nerve fibers arriving through the communicating branch of the nasociliary nerve to the eye. These mixed, short ciliary nerves enter the eyeball around the optic nerve. The second branch of the ophthalmic nerve is the frontal nerve, which bifurcates into the supraorbital nerve to innervate the upper eye lid and the frontal sinus, and the supratrochlear nerve that provides innervation to the forehead and the upper eyelid. Finally, the third branch of the ophthalmic nerve, the lachrymal nerve, innervates the lachrymal gland and some areas of the conjunctiva and the skin of the upper lid. It also incorporates postganglionic parasympathetic nerve fibers from the pterygopalatine ganglion ( Fig. 16.1 ).

A minor part of the sensory fibers from the eye is conveyed, together with the innervation of the conjunctiva and the skin of the lower eye lid, by the infraorbital nerve, a branch of the maxillary nerve, which in turn constitutes the second major branch of the trigeminal ganglion ( Fig. 16.1 ).
Trigeminal sensory fibers end in the epithelia, connective tissue and blood vessels of the lids, orbit, extraocular muscles, ciliary body, choroid, scleral spur, sclera, cornea, and conjunctiva – the retina and lens being the only ocular tissues not receiving direct trigeminal sensory innervation.
1.2
Distribution of sensory nerve fibers within the eye
After penetrating the sclera around the optic nerve at the posterior eye pole, long and short ciliary nerves containing a mixture of sensory, sympathetic, and parasympathetic nerve fibers form a ring around the optic nerve, keeping the lamina cribosa devoid of innervation. Ciliary nerve fascicles travel anteriorly towards the cornea within the suprachoroidal space, giving a few collaterals that provide the sparse innervation of the posterior part of the eye. Along their trajectory to the eye front, these nerve fiber bundles undergo repetitive branching. Some of the nerve fibers innervate the sclera itself, being more numerous in the episcleral tissue; others leave the sclera to enter the choroid, while most nerve filaments continue to innervate the ciliary body, the iris, and the cornea. Most nerve fibers finally form a series of ring meshworks of nerve fibers at the limbus around the cornea – the so-called limbal or pericorneal plexuses.
Nerve filaments entering the choroid are composed of sensory, sympathetic, and parasympathetic axons, that branch extensively forming a dense network in which individual parasympathetic ganglion cells are also found.
Limbal plexus nerves supply the sensory and autonomic innervation of the limbal vessels, the trabecular meshwork and the scleral spur. Nerve bundles also form at the root of the iris, a circumferential plexus that gives origin to the iridal innervation. Finally, a variable number of nerve trunks arising from the limbal plexus enter the corneal stroma (corneal stromal nerves). Despite its comparatively small size, the cornea receives a significant portion of the ocular innervation, presenting a nerve density that is estimated to be 300–400 times higher than that of the human fingers or the teeth.
The majority of the nerves directed to the eye are composed by thin, unmyelinated nerve fibers often incompletely surrounded by Schwann cells, while less than 30 percent present a myelin sheath. All branch extensively and terminate as free nerve endings showing small enlargements (varicosities) along their terminal course. In addition, some encapsulated nerve endings similar to Krause and Meissner bodies have been observed in the choroid, in particular near the ciliary body and the iris, and in the episclera and chamber angle but not in the cornea.
1.3
Architecture of corneal sensory nerves
The corneal innervation is anatomically organized in four levels from the penetrating stromal nerve bundles up to the intraepithelial nerve terminals ( Fig. 16.2A ).

Corneal stromal nerves
The anatomy of nerve bundles radially entering the corneal stroma is quite similar among mammals varying only in their number (6–8 in rat, 15–40 in cat or dog, about 60 in human). Stromal nerves branch immediately after entering the cornea and run within the stroma as ribbon-like fascicles enclosed by a basal lamina and Schwann cells. Myelinated axons included in stromal nerves (about 20 percent of the nerve fibers) lose their myelin sheath within a millimeter after penetrating the stroma ( Fig. 16.2B ). The distal branches of this arborization anastomose extensively, forming the anterior stromal nerve plexus, a dense and complex network of intersecting small and medium-size nerve bundles and individual axons with no preferred orientation laying in the anterior 25–50 percent of the corneal stroma, depending on the species, being most dense in the anterior layers. In contrast, the posterior half of the human stroma and the corneal endothelium are devoid of sensory nerve fibers.
In addition to stromal nerve innervation, a small number of small nerve fascicles originating at the limbal plexus and at the conjunctiva superficially enter the peripheral cornea to innervate the perilimbal and peripheral corneal zones.
Subepithelial nerve plexus
In humans and higher mammals, the most superficial layer of the anterior stromal nerve plexus, located in a narrow strip of stroma immediately beneath Bowman’s membrane, is especially dense and is referred to as the corneal subepithelial nerve plexus, its nerve density generally higher in the peripheral than in the central cornea. Two anatomically distinct types of nerve bundles are distinguished in the subepithelial plexus. One forms a highly anastomotic meshwork made of single axons and thin nerve fascicles located immediately beneath Bowman’s membrane without penetrating it towards the corneal epithelium. The second type consists of about 400–500 medium-sized, curvilinear bundles that penetrate Bowman’s membrane mainly in the peripheral and intermediate cornea, shed the Schwann cell coating, bend at a 90° angle and divide, each into 2–20 thinner nerve fascicles that continue into the corneal epithelium as the sub-basal nerve plexus. A relatively low number of stromal nerves penetrate Bowman’s membrane in the central cornea, which receives most of its innervation from long sub-basal nerves that enter the peripheral cornea directly from the limbal plexus.
Sub-basal nerve plexus
The sub-basal nerve plexus in humans is formed by 5,000–7,000 nerve fascicles in an area of about 90 mm . A sub-basal nerve bundle gives several side branches, each containing 3–7 individual axons. Thus, the total number of axons in the sub-basal plexus is estimated to vary between 20,000 and 44,000.
The sub-basal nerve axons may travel up to 6 mm between the basal epithelial cells and their basal lamina, roughly parallel to one another. The arrangement of a stromal nerve bundle branching into multiple, parallel daughter nerve fascicles, constitutes a unique neuroanatomical structure characteristic of the cornea and is termed an epithelial leash. Epithelial leashes are constituted by up to 40 individual unmyelinated straight and beaded nerve fibers of variable diameter (0.05–2.5 microns). Nerve fibers in adjacent leashes interconnect repeatedly such that they are no longer recognizable as individual leashes, forming finally a relatively homogeneous nerve plexus. In vivo confocal microscopy has shown that the sub-basal nerve plexus forms a whorl-like, spiral pattern of nerve fibers, the center of which is called the “vortex” ( Fig. 16.2C ). In humans it is located 2–3 millimeters inferior and nasal to the corneal apex. The mechanisms that govern the formation and maintenance of this spiral pattern remain unknown. However, as basal corneal epithelial cells and sub-basal nerves appear to migrate centripetally in tandem, it is possible that basal epithelial cells derived from limbal stem cells migrate centripetally in a whorl-like fashion towards the corneal apex in response to a chemotropic guidance, to electromagnetic cues, and/or to population pressures; and the sub-basal nerves that occupy the narrow intercellular spaces between these migrating cells would be pulled along their trajectory. An alternate possibility is that sub-basal nerves would develop their whorl-like orientation independently of epithelial cell dynamics, providing in turn a structural scaffolding that directs epithelial cell migration.
Despite their considerable branching, most stromal and subepithelial nerve bundles pass uninterrupted through the stroma to reach the corneal epithelium and apparently do not provide functional innervation to stromal tissue. However, a small proportion of corneal nerve fibers appear to travel downwards to terminate in the stroma as expanded structures resembling free nerve endings.
Intraepithelial nerve terminals
From the sub-basal nerves running horizontally through the basal epithelium, single fibers split off and turn 90° vertically as a profusion of thin, short, and beaded terminal axons ascending between the epithelial cells, often with a modest amount of additional branching, up to the more superficial layers of the corneal epithelium ( Fig. 16.2D,E ). Intraepithelial fibers end as free nerve endings, appearing as prominent, bulbous terminal expansions that appear morphologically homogeneous when visualized using optical or electron microscopy, though immunocytochemical staining reveals differences in the expression of neuropeptides and other neurotransmitters, suggesting a functional heterogeneity. At the ultrastructural level, corneal nerve endings contain abundant small clear vesicles, perhaps filled with excitatory amino acids, and large, dense-cored vesicles containing neuropeptides such as calcitonin gene-related peptide (CGRP), substance P, and/or other peptides. They also contain mitochondria, glycogen particles, neurotubules, and neurofilaments. The nerve terminals are located throughout all layers of the corneal epithelium, extending up to a few microns of the corneal surface, but being especially numerous in the wing and basal cell layers. Occasionally, epithelial cell membranes facing the nerve terminals show invaginations that may eventually completely surround the nerve ending. This intimate relationship raises the possibility of bidirectional exchange of diffusible substances between both structures. The intimate contacts also allow nerve endings to detect changes in epithelial cell shape or volume, such as those produced by ocular surface desiccation or swelling.
The innervation density of the corneal epithelium is probably the highest of any surface epithelium. Although the actual number of corneal nerve endings remains a matter of speculation, considering that each sub-basal nerve fiber gives at least 10–20 intraepithelial nerve terminals, it is reasonable to speculate that the human central cornea contains approximately 3,500–7,000 nerve terminals/mm 2 . This rich innervation provides the cornea with a highly sensitive detection system, and it has been hypothesized that injury of a single epithelial cell may be sufficient to trigger pain perception. Nerve terminal density, and thus, corneal sensitivity, are higher in the central cornea and decrease progressively when moving towards the periphery. Similarly, corneal sensitivity and nerve density decrease progressively as a function of age and in several ocular pathologies.
An individual stromal axon entering at the corneoscleral limbus undergoes repetitive branching and eventually travels across as much as three-quarters of the cornea before terminating. As a result, individual receptive fields of corneal sensory fibers range in size from less than 1 mm 2 to as much as 50 mm 2 and may cover up to 25 percent of the corneal surface. The extensive branching also explains the significant overlapping of receptive fields found in electrophysiological studies of single corneal nerve fibers.
1.4
Central sensory pathways
Sensory information from the eye is carried by trigeminal ganglion neurons to the ventral portion of the ipsilateral trigeminal brainstem nuclear complex (TBNC), to activate ocular sensory second-order neurons located mainly in the intermediate zone between interpolaris and caudalis subnuclei (Vi/Vc), in laminae I-II of the subnucleus caudalis/upper cervical spinal cord (Vc/C1), and in the adjacent bulbar lateral reticular formation ( Fig. 16.3 ). Additionally, few trigeminal neurons innervating ocular and periocular tissues project to the principal nucleus of the TBNC, and a very sparse number are confined to a few locations along the ventral border of the pars oralis and interpolaris of the spinal trigeminal nucleus. Second-order ocular neurons located in the Vi/Vc area project to different places in the central nervous system including brainstem regions as the superior salivatory/facial motor nucleus and the contralateral thalamus. Neurons receiving corneal information have been identified in the thalamic posterior nucleus and the zona incerta. These thalamic neurons project in turn to primary (SI) and secondary (SII) somatosensory cortical areas responsible for pain sensations and reactions. Non-visual sensory input from the eye is represented in contralateral cortical SI areas 3b and 1 ( Fig. 16.3 ), located together with those that represent nose, ear, and scalp. Projections to the insula and to the cingulate and prefrontal cortex provide the neural substrate for the affective and cognitive components of sensations evoked by eye stimulation.

2
Development and remodeling of corneal innervation
2.1
Development of corneal nerves
The cornea lacks sensory innervation until the fifth gestational month, when corneal nerve endings appear. During development, sensory axons first form the nerve ring around the cornea (the limbal nerve plexus) and subsequently axons grow radially into the corneal tissue ( Fig. 16.4A ). The molecular signals controlling ocular nerve growth remain unknown, though several guidance molecules may regulate the process. Corneal and lens-derived semaphorins appear to mediate the initial repulsion of trigeminal sensory axons from the cornea, thus inducing the proper formation of the pericorneal nerve ring meshwork, and also contribute to the positioning of a subset of axonal projections in the choroid fissure, that form a ventral plexus from which the iris innervation is later supplied. Upon completion of the pericorneal nerve ring, sensory nerves enter the cornea as bundles, at uniformly spaced sites around its entire circumference and begin to extend radially inside the cornea, first innervating its periphery and then the entire stromal surface. The depth at which nerves enter the corneal stroma correlates with the area of the cornea that they will innervate, so that the deepest stromal nerves innervate almost the entire corneal surface, whereas the more superficial nerves which enter the stroma nearest to the epithelium mainly supply the periphery.

The uniform separation between corneal nerve bundles during embryogenesis seems to be due to the release of neuro-repulsive factors from pioneering growth cones as they leave the limbal nerve ring and enter the corneal stroma, keeping nerve bundles growing relatively straight towards the center of the cornea as centripetal radii and separated one from another. The extension or radial innervation within the cornea is produced by bifurcation of nerve fascicles in successive, concentric zones, thus suggesting that the position and orientation of intracorneal nerves is determined by the intra-corneal milieu and the migration of epithelial cells. Stromal composition and neurotrophic factors released from corneal epithelium also participate in the regulation of nerve density and orientation of corneal nerves during development.
2.2
Dynamic remodeling of adult corneal innervation
Corneal nerves are subjected to a remodeling in the adult cornea throughout life. Deep stromal nerve fiber bundles maintain a relatively constant position and configuration within the cornea while the corneal sub-basal nerve plexus, and especially intraepithelial nerve terminals, experience an extensive rearrangement ( Fig. 16.4B ; Box 16.1 ). Time-lapse, in vivo confocal microscopic examination of living human eyes reveals that the human sub-basal corneal nerve plexus is a dynamic structure with a slow but continuous centripetal movement (5–15 microns/day) that changes the whorl-centered plexus in a 6-week period. Intraepithelial nerve endings are subjected to faster morphofunctional changes; their continuous remodeling follows the long-term, sub-basal nerve reconfiguration. The continuous shedding of corneal epithelial cells also strongly influences the rearrangement and dynamics of epithelial nerve terminals.
The continuous remodeling of corneal nerves may be disturbed by an altered corneal architecture or prolonged wound healing. Abnormal patterns in sub-basal nerve organization have been observed following corneal epithelial pathologies such as basement membrane dystrophy, recurrent erosions, or dry eye.
Reorganization of differentiating corneal epithelial cells migrating up to the most superficial layers of the corneal epithelium induces changes in intraepithelial nerve ending architecture that occur in less than 24 hours. The molecular mechanisms responsible for this continuous rearrangement of corneal nerves are unknown. Adult corneal sensory nerves retain the ability to respond to semaphorins, which inhibit nerve sprouting both in transected and intact nerves. In several mammalian species, corneal nerve terminal density decreases with age, and this may be the basis of the decreased corneal sensitivity reported in the elderly.
2.3
Regeneration of injured corneal nerves
Adult corneal nerves retain the ability to regenerate following injury. Nevertheless, after damage or transection, the morphology and functional properties of corneal nerves change substantially. Nerve processes distal to the site of lesion degenerate, whereas central stumps start to regenerate, finally producing a nerve pattern rather different from the original corneal nerve architecture. The regenerative process takes place in several stages ( Fig. 16.4C ). When corneal nerves are cut, the denervated area is first invaded by sprouts of adjacent, intact nerve fibers. Later, the central stump of injured axons starts to regenerate, forming microneuromas from which newly formed sprouts begin to develop, while the early branches from intact fibers begin to degenerate. It may be that interruption in the injured nerve terminals of the uptake of signal molecules such as nerve growth factor (NGF) produced by corneal cells, which are centripetally transported along the parent axon to the neuron’s soma to regulate gene expression, causes morphological and functional changes in regenerating neurons.
3
Functional characteristics of ocular sensory innervation
3.1
Trigeminal ganglion neurons
Electrophysiological studies of ocular sensory neurons have been centered mainly on extracellular recordings of the propagated impulse activity in the peripheral axons of trigeminal ganglion neurons, in most cases nerve fibers innervating the cornea and bulbar conjunctiva. Ocular sensory neurons innervating the cornea and uveal tissues possess in general thin myelinated (Aδ) axons (conducting action potentials at velocities between 3–15 m/s) or unmyelinated (C) axons, which conduct at less than 2 m/s. A reduced number of neurons with thick myelinated axons (Aβ) provide innervation to the limbus, lids, and conjunctiva.
3.1.1
Sensory fibers of the cornea and conjunctiva
As in other parts of the body, the response levels of the peripheral endings of sensory axons to different modalities of physical and chemical stimuli have been used to distinguish various functional classes of sensory fibers innervating the various eye tissues ( Fig. 16.5 ).

Polymodal nociceptors
About two-thirds of the sensory fibers innervating the cornea and the bulbar conjunctiva are activated by physical and chemical stimuli of intensity within the noxious or near noxious range, including mechanical forces, heat, intense cold, exogenous chemical irritants and a large variety of endogenous molecules released by tissue injury. Accordingly, they were named polymodal nociceptors ( Figs 16.5 & 16.6 ). Most of them are unmyelinated while a small portion, depending on species, belongs to the group of thin myelinated (Aδ) nerve fibers. Their receptive fields are round or oval, are usually large, often covering up to one-quarter or more of the cornea, and may extend several millimeters beyond the cornea onto the adjacent limbus and bulbar conjunctiva ( Fig. 16.5 ). The large size and extensive overlapping of adjacent receptive fields of polymodal nociceptors, coupled with convergent mechanisms in the central nervous system, explain why stimuli of the corneal surface are poorly localized.

Corneal polymodal nociceptors respond to their natural stimuli with a continuous, irregular discharge of nerve impulses at a frequency roughly proportional to the magnitude of the stimulus, which persist as long as the stimulus is maintained, thus codifying its intensity and duration. Occasionally, the nerve impulse discharge overpasses the duration of the stimulus (post-discharge). All polymodal nociceptors respond to mechanical stimuli and to temperatures over 39–40°C ( Fig. 16.6A:ii,iii ). About half of the polymodal fibers in the cat also develop a low-frequency response with corneal temperature reductions below 29°C. Polymodal nociceptors are additionally excited by many chemical agents. Acidic solutions (pH < 6.5) ( Fig. 16.6A:iv ) or gas jets containing increasing concentrations of CO 2 (the carbonic acid formed at the corneal surface drops the local pH) evoke robust impulse discharges in corneal polymodal nociceptors. The sensitivity of polymodal nociceptors to acidic stimulation with CO 2 has been used for corneal esthesiometry. A large number of endogenous chemicals (inflammatory mediators) also activate polymodal nociceptors (see below). Expression of different membrane transduction molecules provides polymodal nociceptors with the ability to respond to stimuli of different quality. These include various members of the TRP ion channels superfamily: (a) TRPV1, gated by heat, protons, endogenous mediators and external chemical irritants; (b) TRPA1, that responds to a large number of volatile irritant chemicals; (c) TRPV2 and TRPV3, which are excited by high temperatures. Additionally, polymodal neurons express ASIC channels, which respond to acid and endogenous mediators, while various types of stretch-activated, mechanosensory channels mediate the mechanical responsiveness ( Fig. 16.5C ).
Mechano-nociceptors
Around 15–20 percent of the nerve fibers innervating the cornea respond only to mechanical forces of near-noxious intensity. Accordingly, they were classified as mechano-nociceptors. All the axons of this class of receptor are thinly myelinated (Aδ). The receptive fields are generally round and of medium size, covering about 10 percent of the corneal surface ( Fig. 16.5 ). The force required to activate corneal mechano- and polymodal nociceptors (that is, their mechanical threshold) is around 0.6 mN – about 10 times lower than the force required to activate the equivalent fibers in the skin. This is possibly due to the proximity of corneal nerve terminals to the surface and to the absence of a keratinized surface epithelium in the cornea. Mechano-nociceptors fire only one or a few nerve impulses in response to brief or to sustained mechanical stimuli ( Fig. 16.6A:i ). Therefore, corneal mechano-nociceptors have a limited capacity to codify the intensity and duration of the stimulus and probably serve mainly to signal the presence of noxious mechanical stimuli (touching of the corneal surface, foreign bodies, etc.), presumably being responsible for the acute, sharp sensation of pain produced by sudden mechanical insults.
Cold thermal receptors
About 10–15 percent of corneal nerve fibers discharge spontaneously at the resting temperature of the corneal surface (around 33°C), and increase their firing rate when this temperature is decreased while they are transiently silenced upon warming. Cold receptor fibers have small receptive fields (around 1 mm diameter) located all over the corneal surface but more abundant in the peripheral areas ( Fig. 16.5 ). Corneal cold-sensitive thermal receptors increase their firing rate as soon as the temperature of the cornea drops, which occurs, for example, during evaporation of the corneal surface tear film, application of cold solutions or blowing of cold air on the cornea. In mammals, corneal cold receptor fibers are able to detect small falls in temperature (0.1°C or less) and encode the final temperature in its static impulse frequency. Small corneal temperature reductions are perceived as a sensation of innocuous cooling with a variable irritative component. It is possible that corneal cold thermal receptors contribute to ocular dryness sensations when excessive evaporation reduces corneal temperature. Cold sensitivity of TG ocular neurons depends critically on the expression of TRP8 ion channels. Corneal cold receptors of TRPM8 null mice remain silent and do not respond to cooling. Basal tearing flow in these animals is reduced to half, while the tearing response to ocular surface irritation, which is mediated by polymodal nociceptor stimulation, remains normal. This support the interpretation that this type of fiber is involved in signaling dryness of the ocular surface and contribute to regulate basal tearing flow.
“Silent” nociceptors
The existence in the cornea of nociceptor fibers that are insensitive to all types of stimulus when the tissue is intact but become excitable to mechanical, chemical, or thermal stimuli after a local inflammation develops (“silent” nociceptors) was suggested by McIver & Tanelian. Although experimental evidence for their presence in the cornea is indirect, silent nociceptors have been identified in many somatic tissues, particularly in humans – where they seem to play an important role in inflammatory pain. Therefore it is conceivable that they also exist in the cornea.
3.1.2
Sensory fibers of the sclera, iris, and ciliary body
Although the functional properties of sensory nerve fibers innervating the conjunctiva and the sclera have not been studied in great detail, the same main functional classes of sensory afferents as in the cornea, i.e. polymodal nociceptors, mechano-nociceptors, and cold receptors, have been identified in the sclera, iris and ciliary body, and the bulbar conjunctiva ( Fig. 16.5 ). Mechanosensitive and polymodal nociceptor fibers of the sclera, uvea, and cornea are readily activated by externally applied pressure but only transiently by sudden increases of intraocular pressure up to 100 mmHg, which explains the absence of pain in most forms of glaucoma. However, combination of high intraocular pressure with inflammation, as occurs in congestive glaucoma, possibly causes nociceptor sensitization and a more sustained nociceptive inflow from ocular polymodal nociceptors to the brain, producing the intense pain characteristic for this disease (see below). On the other hand, a small number of low threshold, myelinated mechanosensory nerve fibers responding to moderate changes of intraocular pressure have been identified functionally. They may correspond to axons of the encapsulated nerve endings found in the chamber angle and scleral spur and may be associated with neural regulation of the intraocular pressure.
Cold-sensitive nerve fibers with response properties similar to thermal receptors present in the cornea and conjunctiva are also found in the iris and in the posterior sclera. Cold receptor endings in these locations are not exposed to environmental temperature changes but are close to blood vessels and may serve to detect choroidal and retinal blood flow changes, thus contributing to reflex blood flow regulation rather than to the production of conscious thermal sensations or tearing regulation.
3.1.3
Ocular trigeminal ganglion neurons
The cell bodies of TG neurons innervating the eye are heterogeneous not only in their expression of different transducing channels and their conduction velocity but also in their passive and active membrane properties, thus presenting different impulse firing characteristics. When recorded intracellularly, corneal mechanosensory neurons associated to thin myelinated fibers exhibit fast and short-lasting action potentials, possibly due to the expression of tetrodotoxin-sensitive Na + channels, while Aδ and C polymodal nociceptive neurons are rich in tetrodotoxin-resistant Na + channels and present slower and longer action potentials. Finally, a small population of neurons with a high excitability and very high input resistance has been identified as cold-sensitive neurons.
3.2
Central pathways
Most ocular neurons found in the trigeminal subnucleus caudalis are mechanoreceptive neurons responding only to noxious ocular stimulation or to stimulation of the cornea and periocular skin. A modality-specific distribution of corneal neurons within the trigeminal complex has been proposed. Neurons of the interpolaris and caudalis subnuclei responded to all modalities of stimuli while those within the superficial laminae of the subnucleus caudalis and the cervical cord transition responded only to heat and chemical irritation, suggesting that the input to this region is restricted to TG polymodal nociceptor neurons.
Second-order ocular neurons project to the thalamus and brainstem regions as the superior salivatory or facial motor nucleus – depending on their peripheral input. They participate in several trigeminal-evoked blink reflexes, like the corneal blink reflex, in which corneal afferent information projects to orbicularis oculi motor neurons responsible for lid closing. Likewise, a population of neurons specifically inhibited by wetting and excited by drying of the ocular surface may be involved in reflex tearing and fluid homeostasis of the ocular surface. We can speculate that these neurons receive at least part of their input from peripheral cold fibers innervating eye surface tissues.
Ocular neurons with large receptive fields have been identified in SII areas, where a systematic representation of the face including the eye, and the rest of the body has also been described. Cortical processing of ocular sensory information is sustained by reciprocal interactions between cortical and thalamic areas, and also by a direct modulation of the pre-thalamic relays. So, processing of nociceptive input at the ocular neurons of the TBNC is modified by activation of descending pathways from the pontine parabrachial area and the nucleus raphe magnus mediated by GABA(A) receptors.
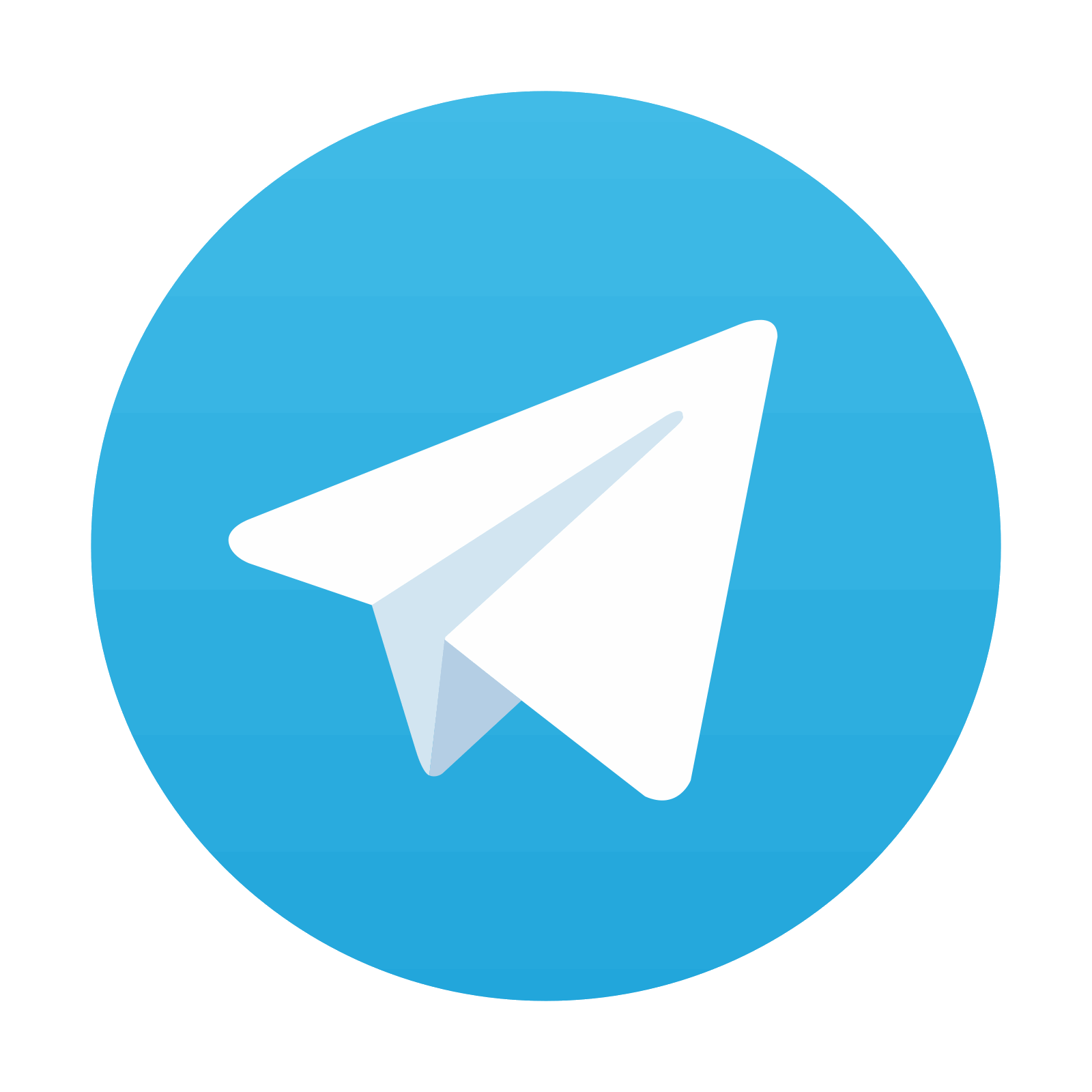
Stay updated, free articles. Join our Telegram channel
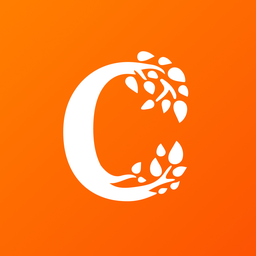
Full access? Get Clinical Tree
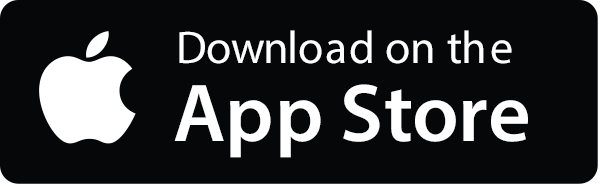
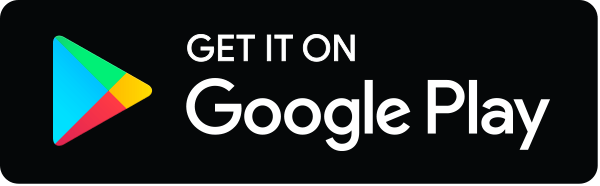