Keywords
Radiation, re-irradiation, fractionation, brachytherapy, simulations


Marie Skłodowska-Curie was fascinated by Becquerel’s findings and, along with her husband Pierre, initiated her landmark work on radioactivity, leading to the discovery and isolation of radium and polonium (a breakdown product of radium). Pierre Curie validated Becquerel’s radiobiological experiment by deliberately producing a radium burn on his own forearm. In 1903 Pierre and Marie Curie and Antoine Henry Becquerel were awarded the Nobel Prize in physics for “joint work concerning investigations of the radiation phenomena described by Henri Becquerel.” Marie Curie received a second Nobel Prize in chemistry in 1911, “in recognition of her services to the advancement of chemistry by the discovery of the elements radium and polonium, by the isolation of radium and the study of the nature and compounds of this remarkable element.” Marie Curie’s contributions included the standardization of radioactivity by quantifying the effects of accurately weighed quantities of pure radium salt in 1911, which continues to serve as the standard to determine the amount of radioactivity in each source.
During the next few decades, improved understanding of radiobiology led to the realization that radiation response is dependent on oxygenation and that fractionation of the radiation dose is required for improved efficacy and better tolerance. In the latter half of the 20th century, new sources of ionizing radiation were discovered, and treatment delivery systems increased in sophistication. In the past 20 years, computerized treatment planning and delivery systems have become the standard of care.
Cell death resulting from ionizing radiation can occur through different mechanisms. The most common cause of cell death is deoxyribonucleic acid (DNA) damage leading to double-stranded breaks. Radiation-induced DNA damage occurs either directly or indirectly by the generation of highly reactive free radicals. The living cell can repair many of these radiation-induced DNA breaks, particularly single-stranded breaks, but tumors cannot, eventually leading to cell death. This damaging effect of radiation may not be evident immediately, but it occurs when the cell attempts to divide. Clinically, the effect of radiotherapy depends on the complex interaction of a multitude of factors. The therapeutic efficacy of ionizing radiation in tumors at most head and neck sites has been well documented. Although control and cure of the disease should be the paramount considerations in choosing the type of therapy, these factors must be balanced against the functional compromise and impact on quality of life. As always, a multidisciplinary approach with close cooperation, not only among the treating team but also with the patient and the family, is crucial in choosing therapeutic interventions. The key factors that influence choice of treatment are shown in Table 19.1 .
Patient Factors
|
Tumor Factors
|
In general, patients with tumors that require extensive surgical resection with sacrifice of organs such as the larynx or the base of tongue are now considered candidates for organ-preserving approaches with use of chemoradiation therapy, reserving surgery for salvage. Tumors, especially skin cancers, that are located in areas that are technically difficult to reconstruct also may be treated with primary radiation to achieve optimum posttreatment cosmesis. For early-staged tumors (T1 or T2), single-modality treatment (either surgery or radiation therapy) is chosen for both the primary tumor and the neck (limited low-volume neck metastases) if appropriate. For advanced tumors, surgery combined with radiation and/or chemotherapy or primary chemoradiotherapy are the preferred treatment modalities ( Fig. 19.1 ). The principles of radiation therapy presented here are intended to serve as a guide for the surgeon involved in multidisciplinary care of patients with head and neck cancer.

Radiobiology
Teletherapy (External Beam Radiation Therapy)
Therapeutic radiation is delivered by two main methods: (1) electromagnetic radiation (photons) in the form of x-rays and gamma rays and (2) particulate radiation in the form of electrons, neutrons, and protons. Ionizing radiation deposits energy at a constant rate as it travels through matter, defined as linear energy transfer (LET). Each unit of absorbed radiation is called one gray (Gy), which is equivalent to one joule per kilogram of tissue. One Gy is also equal to 100 centi-Gy (cGy) or 100 rads. (The rad [radiation absorbed dose] is the previous name for the absorbed dose unit.) Gamma rays are produced within the nucleus of materials, such as cobalt-60, that undergoes radioactive decay, resulting in the emission of gamma ray photons.
Sparsely ionizing or low LET radiation, such as x-rays or gamma rays, deposit their energy less densely per unit length of tissue. On the other hand, high-LET or densely ionizing radiation, such as neutrons, is composed of particles of higher mass that deposit their energy very densely per unit length of tissue. Equal doses of radiation of differing LET do not produce the same biological response in tissues. The relative biological effectiveness (RBE) is a measure of the ability of radiation with different LETs to produce the same biological effect under the same conditions. A 250-kV x-ray beam generally is used as the reference source for comparison. For example, if a 50% cell kill is achieved with 600 cGy of 250-kV x-rays and 200 cGy of neutrons under similar experimental conditions, the RBE of neutrons is 3. In other words, the test radiation (neutrons) is three times as effective as the reference (x-rays). The depth of penetration required is the main criterion used in choosing which energy or teletherapy unit to use. Superficial (40–100 kV) and orthovoltage (250 kV) x-rays have a limited range of penetration and are effective for more superficial lesions, as is electron particulate radiation. Supervoltage (1.25 MV, cobalt-60) gamma rays and megavoltage (4–25 MV, linear accelerator) x-rays, on the other hand, are more penetrative and are used for deep-seated tumors ( Fig. 19.2 ).

Sources used for teletherapy include photons, electrons, neutrons, protons, α particles (i.e., helium atoms, uranium, thorium, radium, and radon), and heavy charged ions (i.e., carbon, neon, silicon, and argon). In patients with head and neck cancer in whom tumors are not deeply seated, the 6-MV photon is used most commonly. Very superficial skin lesions can be treated with electrons and, depending on the size and depth, different energy sources can be used. For recurrent tumors, brachytherapy can be used. Examples of energy sources for brachytherapy are cesium-137, iridium-192, iodine-125, gold-198, americium-24, palladium-103, samarium-145, and ytterbium-169. The typical amount of electromagnetic radiation energy used to treat head and neck cancer is 6 MV.
For particulate radiation, electrons are commonly used to treat head and neck cancers. Electrons are produced in linear accelerators and generally range from 6 MeV to 20 MeV, depending on the depth of penetration desired. Specific examples of the use of electrons in the head and neck region include treatment for skin cancers and sites in proximity to the spinal cord. Neutrons are produced by cyclotrons and can have a maximum energy of 50 MeV. Although they have no dose distribution advantages over photons, neutrons have a higher RBE. Although this higher RBE may be an advantage in treating malignant cells, potentially major complications can result because of their effect on normal tissues. At the present time, their role is primarily in the treatment of unresectable primary and recurrent salivary gland tumors. Due to limited availability, neutron therapy is not routinely recommended in the treatment of head and neck cancer.
Until recently, proton therapy was not available in most institutions. However, due to technologic advances that have allowed for reduction in cost and downsizing of treatment hardware, many proton therapy centers have now opened or are under development. Use of this technology is becoming more widespread and being advocated with the goal of further minimizing normal tissue toxicity, potentially minimizing the risk of the development of second primary tumors as a result of radiation injury to normal tissues and allowing for safer delivery of higher radiation doses to the tumor volume. Protons are positively charged atomic particles that are also produced in cyclotrons. Although protons and photons have roughly equivalent RBE, the characteristic dose distribution of protons allows significantly better control of the depth of penetration. This control can be a major advantage in some situations, such as the treatment of tumors at the base of skull, well-lateralized tumors that only require unilateral treatment of the head and neck, or tumors that have previously been treated with photon radiation. While numerous dosimetry studies have demonstrated superiority in dose distributions with proton plans, recent retrospective data have also suggested improvements in toxicity among well-selected patients. Multiple clinical trials are ongoing to investigate the toxicity profile and appropriate patient populations that stand to benefit most from proton therapy.
During the past several decades, treatment delivery schemes have also evolved. When sophisticated radiation machines were not available, tumors often were treated with a single radiation port using superficial x-rays. However, the depth of penetration of superficial x-rays to a centrally located tumor was not sufficient. With machines that allowed deeper delivery of radiation and the need to ensure delivery of an adequate dose to centrally located tumors, parallel opposed radiation portals were used more often. Wedge pairs also were used to ensure delivery of an adequate dose to the ipsilateral tumor while sparing the contralateral normal tissue.
The introduction of conformal radiation therapy (CRT) followed by intensity-modulated radiation therapy (IMRT) in the early 1990s further minimized the radiation dose to surrounding normal tissues. With advances in computer technology, IMRT has the unique ability to minimize the dose delivered to all surrounding normal tissues while delivering a therapeutic dose to the tumor. Image-guided radiation therapy (IGRT) ensures daily accurate tumor localization while delivering the complex IMRT treatment plans for a given tumor. This involves two-dimensional or three-dimensional images that are acquired at the treatment machine to ensure accurate patient positioning and delivery of the intended radiation plan.
The percentage of cells that survive a given dose of radiation depends on many factors: the type of radiation (low LET versus high LET), dose size, the use of radiosensitizing systemic therapy, environmental conditions (oxygenated versus hypoxic tissue), and underlying tumor biology. The sensitivity of a cell to radiation also depends on its phase in the cell cycle. Cells are most sensitive to radiation-induced damage during the G2 and M phases of the cycle. A tumor consists of cells that are in different phases of the cell cycle and are dividing asynchronously. After exposure to ionizing radiation, surviving cells undergo partial synchronization as a consequence of G2 arrest, which delays cells in a more radiosensitive phase.
Another important factor that affects the ability of ionizing radiation to cause biological change is the amount of oxygen present in the tissue environment; cells in a 100% oxygen environment are three times more radiosensitive compared with completely anoxic cells. Malignant cells within the center of bulky tumors are relatively hypoxic and therefore are relatively radioresistant by virtue of the fact that they are more than 150 µm away from a blood vessel, which is the maximum diffusing distance of oxygen from a capillary. With the delivery of each fraction of radiation therapy, the better oxygenated cells closer to the periphery of the tumor are preferentially killed and, as the tumor shrinks, the centrally located hypoxic cells are brought closer to the blood vessels. This progressive reoxygenation of certain tumor areas may take a few hours or up to a few days to occur. Advanced imaging techniques such as [18F]fluoromisonidazole positron emission tomography (f-miso PET) are under investigation to measure changes in hypoxia during the course of radiotherapy.
Clones that can proliferate during a course of fractionated radiation therapy exist within tumors as well as normal tissue. Proliferation of these cells within tumors can reduce the net effect of treatment and cause local failure. Conversely, within normal tissues, repopulation during treatment is beneficial to the process of healing. Depending on the cell cycle characteristics of the particular tumor, redistribution can either increase or decrease the percentage of sensitive cells. As previously mentioned, cancer cells are also typically less capable of efficiently repairing DNA damage induced by radiation compared with normal tissues, which also contributes significantly to the selectivity of radiation-induced cell death. Factors such as the size and histology of the tumor and radiobiological characteristics such as cell cycle fractions and repopulation therefore are vital to designing effective treatment and form the basis of techniques such as hyperfractionated and hypofractionated schedules.
Fractionation
The technique of administering radiation therapy in fractions instead of in a single high dose originated in 1927. The underlying principle of fractionation is that a high total dose can be delivered to the tumor while sparing adjacent normal tissue. Generally, the total dose of radiation, the size and number of fractions, and the duration of treatment are determined by the type of tumor and the tolerance characteristics of normal tissue in the treatment field. The conventional fractionation schedule that entails use of 180 to 200 cGy per fraction, one fraction per day, 5 days per week for 6 to 7 weeks for a total dosage of 6500 to 7000 cGy has evolved empirically over many years. Based on both laboratory and clinical research, it is now apparent that the conventional fractionation scheme may not be the best approach for all tumors, particularly in the head and neck region.
Three important areas form the foundation for the evolving use of altered fractionation: (1) tissue response; (2) duration of treatment; and (3) fraction size and number. Acutely responding tissues are rather active in ongoing cellular proliferation. Most tumors (except perhaps prostate cancer, breast cancers, and melanoma) and some normal tissues such as skin, mucous membranes, and gastrointestinal epithelium share this characteristic. These tissues are most affected by the overall treatment duration rather than by the size or number of fractions used. Late-responding tissues have a low proliferative rate and include the spinal cord, brain, bone, and cartilage. These tissues are most affected by the size and number of fractions rather than by treatment duration and therefore are spared by decreasing the dose per fraction of radiation delivered.
Because most tumors consist of rapidly dividing cells, local tumor control is strongly dependent on the overall treatment duration rather than on the size or number of fractions. When squamous cell carcinoma of the head and neck is exposed to radiation, the less radiosensitive cells within the lesion can undergo rapid proliferation approximately 3 to 5 weeks after treatment commences. This accelerated repopulation can overwhelm the ongoing treatment effects of radiation, which ultimately can lead to local failure. The clinical significance of this phenomenon is that even with significant regression of the primary tumor mass, local failure still ultimately could result from proliferation of these resistant clones. Therefore it is essential to complete treatment in as short a time as possible so that accelerated repopulation is minimized, increasing the chance for local control. For this reason, split-course radiation which incorporates a treatment break during the course of radiotherapy is not recommended.
Based on the aforementioned principles, the goal of altered fractionation schemes is to improve the therapeutic ratio by maximizing the tumoricidal effect and minimizing acute and late toxicities while using readily available low-LET radiation. Two major categories of altered fractionation schemes exist: hyperfractionation and accelerated fractionation. They share basic radiobiological principles yet have their own particular features ( Table 19.2 ). Accelerated fractionation is the strategy of choice for rapidly proliferative tumors, whereas hyperfractionation is preferred for slowly proliferating tumors. Hyperfractionation improves the therapeutic ratio primarily through (1) redistribution of tumor cells into more radiosensitive phases as a result of multiple fractions and (2) differential sparing of late-responding normal tissues because of a decrease in the size of the dose per fraction. Accelerated fractionation is based on the concept that the shortened overall treatment time would reduce the opportunity for accelerated repopulation effectively.
HYPERFRACTIONATION | ACCELERATED FRACTIONATION | HYPOFRACTIONATION |
---|---|---|
|
|
|
A randomized study by radiation therapy oncology group (RTOG) 90–03 evaluated the use of low-LET radiation alone with four fractionation schemes for the treatment of squamous cell carcinoma of the head and neck. Patients included in this trial underwent radiation therapy as a single modality, without the use of chemotherapy. The sites included the oral cavity, oropharynx, hypopharynx, and supraglottic larynx. The stages were limited to III and IV (with no distant metastases); however, the base of the tongue and the hypopharynx subsites included stage II patients as well. The four arms were as follows: (1) conventional fractionation; (2) hyperfractionation; (3) accelerated fractionation with split; and (4) accelerated fractionation with a concomitant boost. A significantly improved 2-year locoregional control and disease-free survival rate occurred with accelerated fractionation with a concomitant boost compared with conventional fractionation and accelerated fractionation with a split. Patients treated with hyperfractionation also had a trend toward improved results. However, a phase III Groupe Oncologie Radiotherapie Tete et Cou cooperative trial did not show a benefit when altered fractionation was combined with chemotherapy. In fact, patients treated with accelerated fractionation with concurrent chemotherapy experienced more toxicities than did patients treated with conventional fractionation with concurrent chemotherapy.
The RTOG 99-14 trial asked the same question about whether chemotherapy given concurrently with concomitant boost radiation can further improve on locoregional control. Because of the encouraging preliminary results, RTOG 01-29 was conducted to answer the question of whether altered fractionation should be used in the setting of chemotherapy. The results of this two-arm prospective randomized trial of more than 700 patients was just recently reported, showing that when chemotherapy is given concurrently with radiation, there is no added benefit of using altered fractionation compared with standard once-daily radiation. Furthermore, the long-term grade 3 to 4 late toxic effects of chemotherapy from RTOG 99-14 with concomitant boost radiation was extremely high at 42%. Gastrostomy tube dependence rates anytime during follow-up, at 1 year, and at 2 years were 83%, 41%, and 17%, respectively. However, it should be mentioned that these patients were treated with older, conventional nonconformal radiation techniques, such as Cobalt 60. Since the introduction of IMRT, which allows for significant reduction in radiation dose to normal tissues, treatment-associated toxicities have improved. Three randomized studies comparing conventional radiation technique versus IMRT for head and neck cancer have indeed shown that there are lower late complications with IMRT. Furthermore, there is no evidence that IMRT causes compromise in locoregional control.
Hypofractionation is the administration of high-dose-per-fraction (HDPF) radiation, in which only one or two fractions are given per week. This technique has evolved for the treatment of malignant melanoma, which generally is perceived as being radioresistant. Conventional fractions of 200 cGy delivered 5 days a week allow normal tissues and tumor cells to recover during the intervals between fractions. Experimental in vitro data have shown that malignant melanoma cells are better at repairing radiation-induced sublethal damage compared with other cells. This finding may explain the long-standing notion that melanoma is intrinsically “radioresistant.” HDPF regimens deliver higher doses of radiation per fraction (600 cGy twice a week or 800 cGy once weekly) with the aim of overcoming the reparative capacity of the tumor cells by increasing the damage per fraction. In retrospective analyses, response rates have been shown to correlate with dose per fraction but not with the total dose delivered. However, a prospective randomized trial (RTOG 83–05) found no therapeutic advantage in a comparison of HDPF (800 cGy once a week up to a total dose of 3200 cGy) and conventional fractionation (250 cGy daily, 5 days a week, for a total of 5000 cGy). Although no therapeutic advantage was seen, the shorter delivery time of HDPF radiation allows earlier initiation of systemic therapies if applicable. Moderately hypofractionated radiation (225 cGy per fraction), however, has demonstrated superior results for early-stage larynx cancers treated with radiotherapy alone and is currently considered the standard of care in this setting. Additionally, a regimen commonly referred to as quad shot , which was originally developed for advanced pelvic tumors, is sometimes applied for palliation of tumors in the head and neck. This involves cycles of a 1480 cGy course of radiotherapy delivered in four fractions over the course of 2 days, which can be repeated multiple times over a period of weeks or months depending on the treatment response. Aside from the demonstrated efficacy of this regimen, it also allows significant advantages in terms of patient convenience in the palliative setting.
Radiation therapy can also be delivered with use of a single fraction. Intraoperative radiation therapy (IORT) is a form of brachytherapy in which radiation is given to the target area while the patient is under anesthesia. It is often used to treat recurrent tumors that have previously been treated with radiotherapy. Stereotactic radiosurgery is a technique whereby radiation is delivered with use of stereotactic principles, primarily established for brain tumors. However, stereotactic body radiosurgery increasingly is being used to treat tumors of the lung, pancreas, liver, spine, prostate, and other sites. Gamma knife radiosurgery using cobalt sources has limited utility, because it is used to treat brain metastases or benign conditions such as trigeminal neuralgia. Gamma knife radiosurgery can be used to treat isolated skull base metastasis.
Dose, Ports, and Beam-Modifying Devices
Although physicists measure a radiation dose as the amount of ionization that it produces in air (roentgens, or R), it is the absorbed radiation dose in tissue that is of clinical relevance. Because performing this measurement is difficult and impractical, the rad is a derived value. One roentgen of exposure in air is equal to approximately a 0.95 cGy absorbed dose in tissue. In contemporary radiation oncology, a dose is expressed as the amount of energy absorbed per unit mass of absorbing material, or cGy, which is equal to 100 ergs absorbed per gram.
Portals or fields used for directing the beams of treatment are designated depending on the direction of entrance into the patient’s body ( Fig. 19.3 ). Port films are critical for verifying the field of treatment and are obtained at the start of treatment and at daily or weekly intervals thereafter. Treatment units produce fields that are square or rectangular and deliver a consistent dose across a plane perpendicular to the central ray of the beam. Therefore the beam needs to be shaped and modified according to the configuration of the anatomy and the shape of the tumor.

Shielding blocks, made from Cerrobend lead, generally achieve this goal because their shape and thickness can be varied according to the energy of the beam. The shape and position of the blocks are verified on the port films before treatment is started. A bolus is a material such as petroleum jelly–impregnated gauze, water bags, paraffin wax, and other devices that have interactions with the radiation beam similar to those of tissue. The bolus generally is used to minimize underdosage of superficially situated tumors and scars. For example, filling the postoperative defect of a maxillectomy with petroleum jelly–impregnated gauze improves the homogeneity of dosing to the target.
Compensators are filters positioned within the head of the treatment unit that modify dose distributions. These filters generally are made of copper, brass, Lucite, or lead. When multiple treatment volumes are oriented adjacent to each other, the isodose curves may overlap, resulting in an increased dose at the junction. Depending on the situation, a junction block or creation of a gap between the two adjacent areas may be necessary, especially over the spinal cord. Wedges function to change the angle of the isodose curve of a particular field relative to the beam axis at a specified depth so that the dose distribution can be evened out.
In the modern era, treatment machines typically utilize multileaf collimators that have the capability of not only static but also dynamic shaping of the beam during treatment, which avoids the need to manually change the blocks required for differing fields. Three-dimensional CRT involves treatment with multiple fields that enter the patient from different directions and are designed to avoid normal organs while delivering the desired dose to the tumor volume. IMRT builds on this approach by shaping the radiation beam continuously during treatment delivery with the use of moving multileaf collimators. IMRT is considered to be “inverse planned,” meaning that the tumor and organ volumes are contoured manually by the physician and computer software is used to generate a radiation plan with optimal characteristics in terms of tumor coverage and organ sparing. Volumetric arc therapy, or VMAT, is a more recent iteration of IMRT that involves continuous delivery and shaping of the radiation beam as the treatment gantry rotates around the patient. This technique allows for significantly reduced treatment times and improvements in dose distribution in some scenarios.
Brachytherapy
In contrast to teletherapy, brachytherapy uses selected radioisotopes and specialized instruments to administer radiation directly to a tumor or its bed. The radiation sources are placed either adjacent to the surface of a tumor mass or tumor bed or inside the tumor itself. The treatment may involve permanent implantation of radioactive sources (e.g., permanent 125 I seeds injected into a recurrent nasopharyngeal mass) or only a temporary exposure, after which the source is removed (e.g., intracavitary insertion of 125 I seeds for a localized recurrent nasopharyngeal carcinoma or temporary interstitial catheter implantation for afterloading with 192 Ir sources for a neck mass or tumor bed).
Brachytherapy radiation travels only a short distance to the desired target region, and its dose intensity falls off rapidly with distance according to the inverse square law. This phenomenon permits a sharp decrease in the dosage to the surrounding normal tissue so that the radiation dose is delivered to a relatively small, well-defined volume. Low-dose-rate (LDR) brachytherapy delivers continuous radiation at a rate of 40 to 200 cGy per hour, whereas high-dose-rate (HDR) brachytherapy delivers in excess of 1200 cGy per hour. In radiobiological terms, LDR brachytherapy can be likened to fractionated radiation with an infinite number of small individual doses. The theoretical advantage of this approach is that it allows redistribution of the tumor cell within the cell cycle, resulting in a greater percentage of malignant cells in the more radiosensitive phases. It also allows time for reoxygenation of hypoxic cells during the treatment and thus results in an increase in their radiosensitivity.
LDR brachytherapy favors late-responding normal tissues relative to tumors, and repopulation does occur, but unfortunately it benefits tumors more than normal tissues. HDR treatments have a higher risk for complications involving late-responding tissues. Therefore HDR brachytherapy needs to be well fractionated to deliver only one to three fractions per week. The isotopes most commonly used in head and neck cancer treatments are 125 I and 192 Ir. The implants can be planar (single plane) or volumetric (more than one parallel plane separated by 1–1.5 cm).
Head and neck tumor sites commonly considered suitable for brachytherapy include the lip, floor of mouth, oral tongue, base of tongue, buccal mucosa, tonsillar region, nasopharynx, skull base, and neck. The size and volume of the primary lesion, the anatomic extent, the topography, adjacent vital organs, any prior therapy, and the medical condition of the patient must be critically evaluated when considering a case for possible brachytherapy intervention. In the oral tongue and floor of mouth regions, T1 and T2 lesions can be treated with brachytherapy alone or in combination with external beam radiation therapy with good results. However, the risk of possible complications from treatment such as soft tissue or bone necrosis must be weighed against consideration of a primary surgical approach with its low complication rate. When the radioactive sources are in close proximity to the gum and bone, the risk of complications (such as bone exposure and necrosis) greatly increases. Other contraindications include an inability to encompass the tumor with adequate margins or inadequate access to the tumor. This form of therapy therefore is ideal as an adjunct to surgery or external beam radiation therapy.
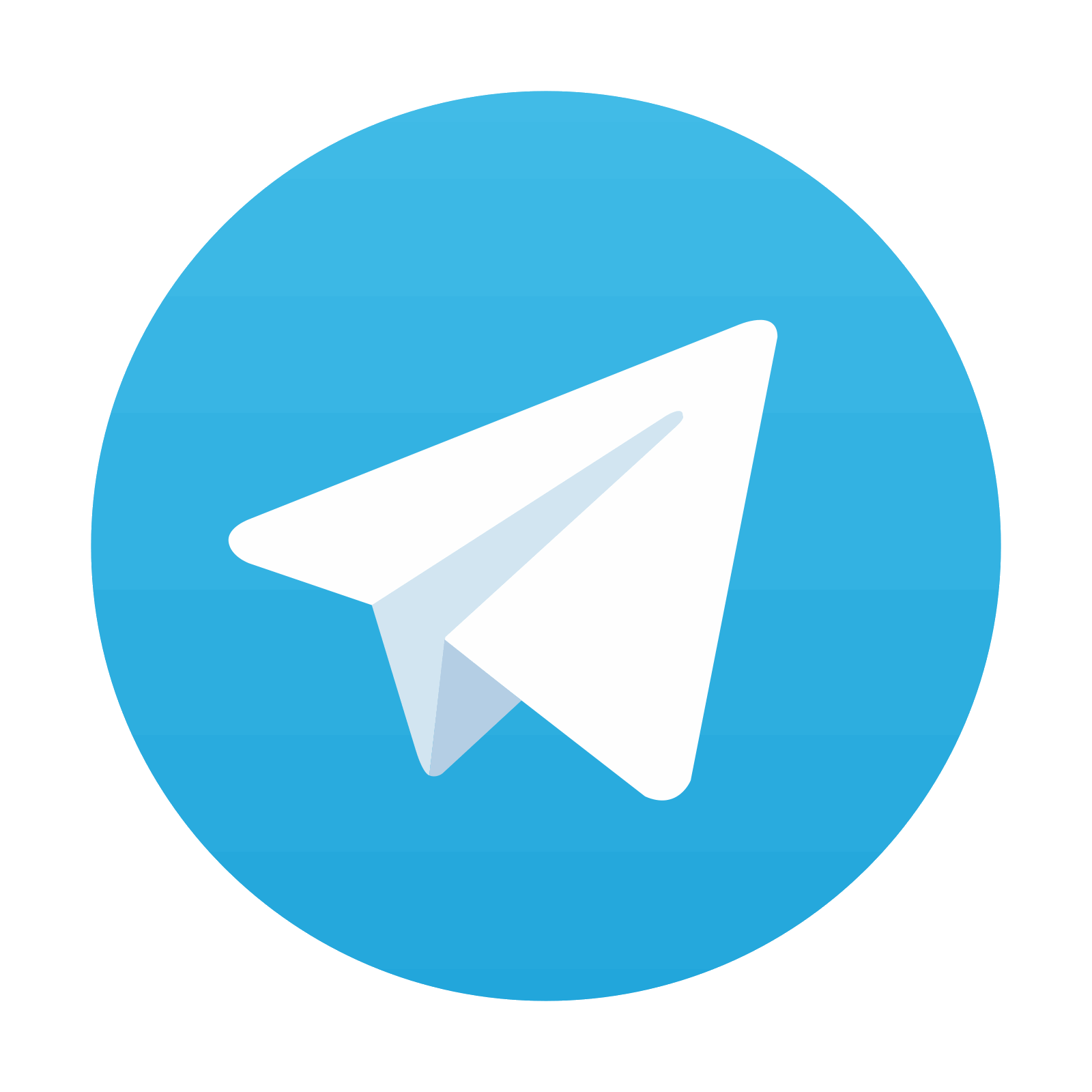
Stay updated, free articles. Join our Telegram channel
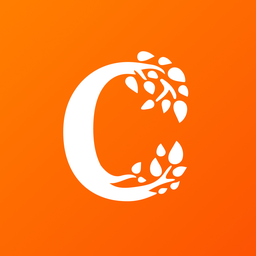
Full access? Get Clinical Tree
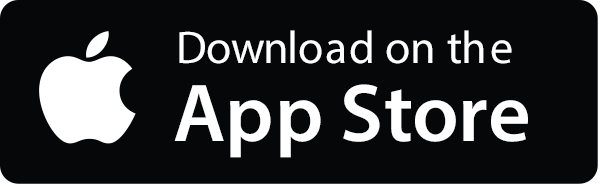
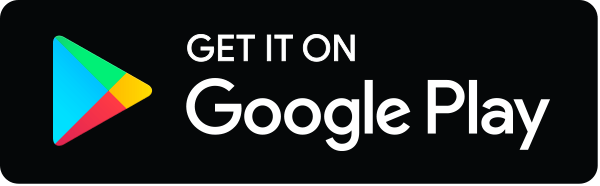