Overview: The primary visual cortex constructs local image features
The visual system provides a description of the location and identification of objects that have survival value to the species. This description must be made in a dynamic world in which our gaze is constantly shifting and in which objects move. To be useful, the description must select items of importance depending on context and memory; in essence the visual system is designed to make useful predictions about visual reality, not to actually represent reality. Implicit in the architecture of the visual system reside mechanisms tuned by evolution and development to optimize useful vision. Beginning in the retina, the visual system selects what is needed to accomplish this goal. The retina contributes to this selection process by eliminating information about absolute light intensity, emphasizing local image contours, and compressing the visual signal information into a manageable size during a manageable time window to be transmitted to the lateral geniculate nucleus (LGN, see Chapter 29 ). The LGN contributes by regulating the flow of visual signals. Primary visual cortex (V1), as we will learn, contributes by coding aspects of local image features including their size, orientation, local direction of movement, and binocular disparity. All of these local descriptions of stimulus quality are critical for the more global and complex identification of objects (“what”) and spatial relations (“where”).
Classically, V1 is described as an area where information provided by the separate LGN channels is combined in different ways before it is sent to extrastriate visual areas for further processing. In order to do its job, V1 must solve the geometry puzzle of representing all stimulus qualities necessary for the subsequent steps of analyses within tissue that maps the outside world, i.e. responds to different parts of the visual field. V1 accomplishes this goal by a division of labor between different layers and different iterated modules within each layer. In this classic view, V1 is pictured as the beginning stage for several sets of serial processes that take place in feedforward hierarchies of visual areas; areas specialized to perform a variety of higher-order tasks. Currently, however, there is growing recognition that V1 does more. V1 does not represent stimulus qualities statically. Via dense and intricate horizontal and feedback pathways, V1 dynamically interacts with higher visual and non-visual areas, as well as the thalamus, and alters its activity and message depending on attention, memory, context, and reward. Therefore, for efficiency, V1 cells bias their messages to emphasize moment to moment relevance. In this chapter we initially provide the reader with a road map to the organization of V1, we show how the anatomical architecture of the V1 sets limits on how visual signals can be processed, and we emphasize the laminar architecture, connections, and cell types. Next we review the traditional view of how receptive field properties are put together in V1 and review the hypothesis that the parallel inputs from the LGN relate in functionally specific ways to output channels of V1 (see also Chapter 31 ). The second to last section then briefly reviews more controversial ideas concerning the functions of V1, including whether V1 is conscious and participates in memory or is modified by reward and attention. The final section of this chapter summarizes key points.
Overview of cortical organization: a general road map
Human V1 ranges in size from 1400 to 3400 mm 2 , is about 2 mm thick, and is located within the occipital lobe extending from the posterior pole along the medial wall of the hemisphere ( Fig. 30.1 ). Like the rest of the cerebral cortex, V1 contains 6 principal layers. V1 is often called striate cortex in recognition of its original identification by an Italian medical student (Francesco Gennari) more than 200 years ago. Gennari observed that if he sliced into the posterior pole of an unfixed human brain and observed the cortical layers of the slice a striking white stripe was visible, known today as the stria of Gennari . The stria of Gennari actually marks one heavily myelinated layer in the middle of the gray matter of V1. V1 also is referred to by several other names including, most commonly, area 17 of Brodmann or primary visual cortex. For convenience we will use the term V1 in this chapter.

Damage to V1 results in a hole (scotoma) or blind spot in one visual hemifield. The location of damage to V1 can be predicted based upon the topographic map of the opposite visual hemifield that is known to exist in this region in all mammalian species. In humans, detailed knowledge about the manner in which the visual field is mapped onto V1 has been obtained from a variety of sources including clinical assessments of damage, results of electrical stimulation, and transcranial magnetic stimulation (TMS). The latter is a non-invasive method used to excite neurons by using rapidly changing magnetic fields to produce weak electrical currents. Both direct invasive electrical stimulation and non-invasive TMS can give rise to the experience of localized phosphenes (flashes of light) experienced by the subject. Other methods of developing functional maps of V1 have used positron emission tomography (PET) and, most commonly, functional magnetic resonance imaging (fMRI). In the latter case the subject is presented with topographically restricted stimuli in different locations in space and the relative activation of V1 measured as a change in blood flow. Knowledge about the retinotopic organization of V1 in humans is relevant not only to the clinical evaluation of damage, but also to research efforts designed to develop visual prosthetic devices involving visual cortical electrical stimulation for individuals with incurable retinal diseases or loss of eyes ( Box 30.1 ). All of the methods used to understand the map of the visual field in V1 are in general agreement showing, as illustrated in Figure 30.1 , that the fovea is represented in the occipital pole and the far periphery is represented in the anterior margin of the calcarine fissure with the upper and lower visual fields being mapped onto its lower (lingual gyrus) and upper (cuneus gyrus) banks, respectively. As in the LGN, the visual field map in human cortex is distorted such that the representation of central vision occupies much more tissue than does peripheral vision. Whether the foveal representation in V1 is expanded over what would be predicted simply by assigning each retinal ganglion cell or LGN cell the same amount of cortical tissue has been the subject of considerable debate. One explanation for this controversy is that the proportion of cortex devoted to central vision has been shown to be highly individually variable, at least in macaque monkeys. The latter finding indicates that the relative amount of tissue devoted to the fovea could be magnified at the cortical level relative to the retina in some individuals but not others.
Given the success of cochlear implants for the deaf, expectations have been high that a prosthetic “eye” could be developed soon. Although great strides have been made, the results so far are not yet optimal. Here we outline progress using V1 as the site for visual prosthetic development. Scientists have known for several decades that electrical stimulation of the visual cortex will cause blind patients to see phosphenes or small spots of light. These spots can appear bright or dim or colored or flickering depending on the level of electrical stimulation. There are a number of problems that need to be overcome to make electrical stimulation of V1 useful as a visual prosthetic device. These include, but are not limited to, the density and location of electrodes implanted in V1, tissue reaction to the electrodes and input devices to translate the visual scene into appropriate patterns of electrical stimulation. The ideal system would have a high density of electrodes activated wirelessly via a light-weight, high-performance camera/computer system appropriate for ambulatory patients.
Thus far, some successes have been achieved using this method by several groups. The most notable successes have been reported by the late Dr. William Dobelle and the Dobelle Institute (Portugal) where a number of patients have been implanted with arrays of electrodes in V1. Although these patients do not experience vision in the normal sense they are able to use the phosphene patterns generated in V1 to identify the outlines of objects and even, in one case (Jens), slowly drive a car around a parking lot.
Layers, connections, and cells of V1: The inputs, outputs, and general wiring
As in other cortical areas, V1 has six main layers that can be identified in a cell stain as shown in Figure 30.2 . These layers contain two main types of cells, pyramidal and stellate, in an 80/20 proportion. The layers and sublayers of V1 have been named in different ways depending on investigator interpretation. The most common laminar scheme is the one adopted by Brodmann. Although Brodmann likely made a major mistake in his interpretation of V1 layers in all primates his scheme is the most accepted so we use it here ( Fig. 30.2 ). As in other areas of neocortex, input from the thalamus ends primarily in the middle layers (IVC of V1), with the superficial layers (above IVC) sending information to other cortical areas and the layers below IVC sending signals to subcortical targets ( Fig. 30.2 ).

LGN inputs
Studies done in anesthetized monkeys have demonstrated that activation of V1 neurons depends completely on input from the LGN, because when the LGN is inactivated, visually evoked potentials in V1 are blocked. LGN axons carrying signals from the left and right eyes and from K, M, and P layers remain segregated at the first synapse in V1 in non-human primates and presumably also humans ( Fig. 30.3 ). The left and right eye segregation of axons from the M and P LGN layers ending in layer IVC of V1 is referred to as ocular dominance columns. These “columns” have been directly demonstrated to exist in humans as shown in the complete reconstruction of the pattern of left and right eye input to V1 in Figure 30.4 . These zebra-stripe-like patterns of monocular segregation from LGN can be revealed in tangential sections of layer IVC of V1 in humans postmortem following long-standing loss of one eye. As shown in Figure 30.4 , the patterns are revealed by a histochemical stain for the mitochondrial enzyme, cytochrome oxidase (CO) which becomes down-regulated in the zones of layer IVC innervated by LGN axons from the missing eye. As mentioned and shown schematically in Figure 30.3 , K, M, and P LGN axons in macaque monkeys also terminate in separate V1 layers or sublayers. M and P axons synapse in sublayers of IVC, and K LGN axons synapse in layers IVA, III, and I. Also, K LGN axons are likely made up of several classes since those that end in different V1 layers appear to come from different populations of LGN cells in macaques. It is noteworthy, however, that the LGN does not project to layer IVA in apes and humans, reminding us that care must be taken in translating data directly from other primates to humans.


The fact that input from the left and right eyes remains segregated at the first synapse in V1 raises an interesting question concerning the retinotopic map. Recall that in the LGN each layer contains a continuous map of the opposite visual hemifield. This means that in cortex there must be two topographic maps, one for each eye, within the same layer, at least at the first synapse from the LGN. This is exactly what was found in layer IV of the macaque monkey using detailed electrophysiological recordings. Tangential recordings made within layer IVC revealed that the segments of the visual field mapped in one ocular dominance column for one eye were also represented in the adjacent column that received input from the other eye. As we will see below, information from the two eyes and from K, M, and P cells is combined in different ways within other layers of V1, hence, most cells in V1 receive a combination of many extrinsic and intrinsic inputs.
Other inputs to V1
Besides the LGN, V1 receives a variety of other modulatory inputs both from subcortical and cortical areas. These inputs include serotonergic, noradrenergic, and cholinergic inputs from the brainstem and basal forebrain nuclei, respectively. The latter inputs show differences in density in the V1 layers, but show a much less specific pattern of innervation than do LGN inputs. Other input sources include the intralaminar nuclei of the thalamus and the pulvinar, both of which send broad projections most heavily to layers I and II of V1. Additionally, there are retinotopically more specific sources of input to V1, including from the claustrum and visual (V) areas 2, 3, 4, & 5 (V4 and V5 are also referred to as the dorsal lateral (DL) and middle temporal (MT) visual areas, respectively). , As a general rule any area to which V1 projects also sends feedback to V1. However, some higher-order visual areas in the temporal and parietal lobes that do not receive direct projections from V1 send axons to V1. With the exception of the claustrum, whose axons overlap with M and P axons in layer IVC, all of the other extrastriate visual inputs to V1 terminate outside of layer IVC.
So why are there so many other inputs to V1 if the main drive comes from the LGN? As with the LGN described earlier, the numerous non-geniculate inputs to V1 regulate which visual signals will be transmitted to higher-order visual areas. An example of the impact that these non-LGN connections to V1 can have has been demonstrated using fMRI neural imaging methods. Using fMRI in humans, it has been shown that topographic regions of V1 can be activated simply by asking normal subjects to imagine (with eyes closed) visual objects within particular areas of the visual field (i.e. in the absence of any direct stimulus to the retina). Additionally, evidence indicates that, in congenitally blind subjects, major rearrangements of connections can take place such that somatosensory inputs can now activate V1, or changes in receptor distribution at the retina can cause rewiring of V1 ( Box 30.2 ). These findings argue that non-LGN inputs can have a strong impact on activity in V1 and that V1 is highly plastic during development.
Cones are silent under dim light conditions. Since the normal human fovea is made up exclusively of cones, humans are blind in the area representing the fovea in dim light when only rods are operating. Using functional magnetic resonance imaging (fMRI) Baseler and colleagues (2002) were able to confirm that the area of V1 representing the fovea is inactive in normal humans under these dim light conditions. As the schematic below shows the fovea is represented over a substantial piece of tissue in cortex (several square centimeters). In other words, a large portion of V1 is presumably idle while you look for your seat in a dark theater or take a romantic moonlight walk.
Interestingly, these same investigators, using the fMRI were able to demonstrate a large-scale developmental reorganization in the human rod monochromats. Rod monochromats are congenitally color-blind individuals that almost completely lack retinal cones. In rod monochromats the foveal representation in V1 becomes reorganized so that it now responds strongly to visual stimuli presented under rod viewing conditions. These findings reinforce earlier work in both humans and animals suggesting that V1 is plastic and capable of large-scale reorganization when inputs are abnormal during development.

Schematic of the activation of V1 for normals ( A and B ) or for rod monochromats ( C ) under scotopic ( B and C ) and photopic (A) conditions. ( D ) represents the central 16 degrees of one visual hemi-field as described in the text. There is a mapping between visual space and cortical space, represented here by the correspondence between the colors in the schematic diagram of the right visual field ( D ) and the colors in the schematic diagrams of the left V1 unfolded and flattened ( A , B , and C ). Under normal lighting conditions the entire visual cortex of normal viewers is activated ( A ). Under scotopic conditions in normals the foveal representation is inactive represented in gray ( B ). In rod monochromats no fovea exists and the cortex is reorganized to represent the rest of visual space ( C ). In ( A ), ( B ), and ( C ) the vertical meridian (VM) runs along the upper and lower edges of the figure while the horizontal meridian (HM) is represented by the dashed horizontal line.
Cell classes and connections within V1
In V1, cells containing glutamate account for the vast majority (~80 percent) with the remaining cells containing GABA. These two main cell classes are morphologically distinct. There are two types of glutamate-containing cells: the spiny stellate cells that occur mainly in layer IV, and the pyramidal cells that occur in all of the layers. Both of these glutamate-containing neurons have a high density of dendritic spines. In contrast to the pyramidal cells, the inhibitory GABAergic cells have few to no spines on their dendrites. The latter are multipolar neurons whose dendritic arbors come in a variety of shapes. Many subclasses of GABAergic interneurons have been identified based also on physiology and the presence of different types of proteins such as different calcium-binding proteins, or various peptides. Although many models have been proposed to explain the role(s) of different types of inhibitory interneurons in V1 there is no general agreement on function for any class.
Connections between layers can be made by both excitatory and inhibitory neurons (see reviews , ). Efforts to trace the overall flow of information in V1 have been hampered by the multiplicity of connections and diversity of cell classes. Using pharmacological manipulations it was demonstrated that layer IVC becomes active first, then the upper layers, followed by the lower layers. Studies of cell responses in slices of visual cortex in monkeys (where photic uncaging of glutamate has been used to stimulate small groups of neurons) have shown that, although connections can be quite precise, there exists tremendous diversity of responses even among cells thought to have common inputs in the same layer. If one attempts to trace anatomical pathways beyond the main input layer IVC, it also is difficult to decipher how separate output pathways are constructed in V1 due to the tremendous opportunity of intermixing; nevertheless, V1 does appear to give rise to distinct output pathways that emerge from somewhat different populations of input cells ( Fig. 30.5 ).

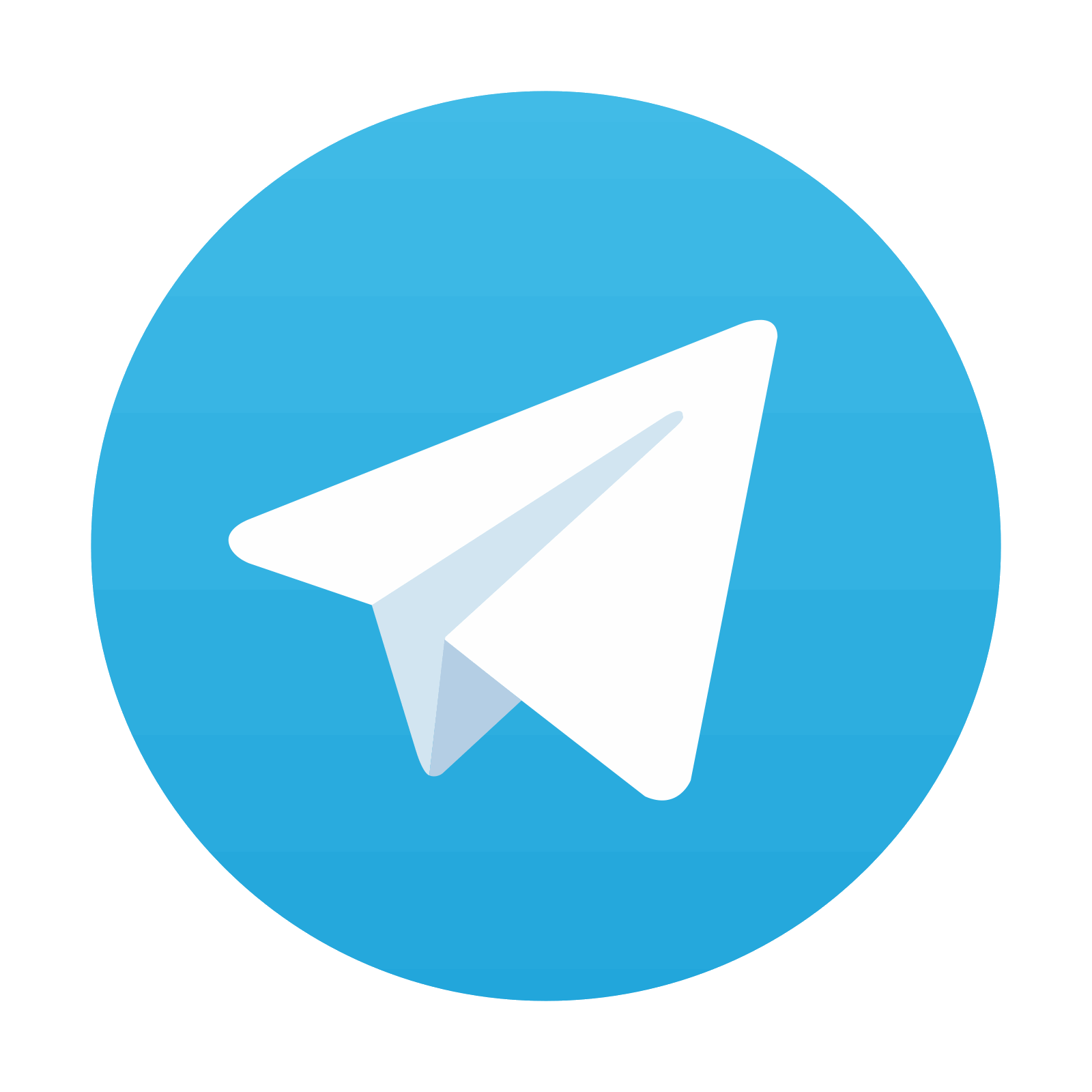
Stay updated, free articles. Join our Telegram channel
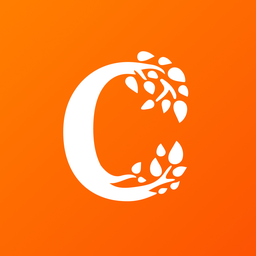
Full access? Get Clinical Tree
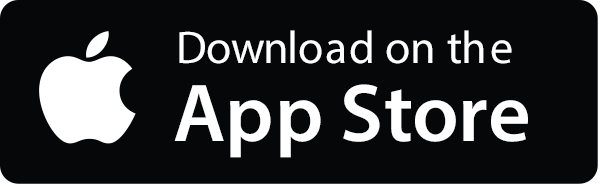
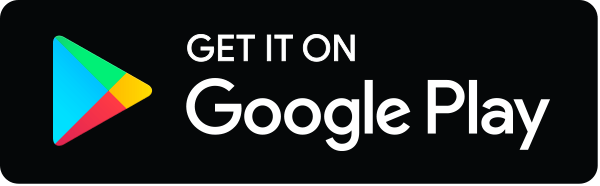
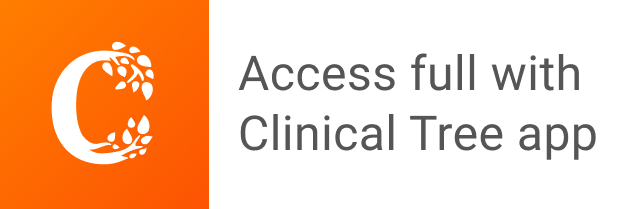