The lateral geniculate nucleus: the gateway to conscious visual perception
Located one synapse from the retina, the lateral geniculate nucleus (LGN) of the thalamus is the main link between eye and cortex. Although the retina connects with other subcortical structures, the route through the LGN is required for conscious visual perception. Connections between retinal ganglion cells (RGCs) and LGN neurons are highly specific and establish channels of visual information that preserve the encoding of visual stimuli within the retina. Rather than transform visual information originating in its RGC inputs, the LGN modulates the transfer of retinal information to cortex based on visual demands, changes in brain state (sleep, arousal), and attention. The LGN modulation by sleep-arousal is thought to be mediated by inputs from the brain stem and the modulation by attention by inputs from the visual cortex. In addition, inputs from the superior colliculus (SC) to the koniocellular layers of the LGN may also contribute to a form of subconscious vision known as blindsight. The purpose of this chapter is to illuminate the structure and function of the LGN by first reviewing LGN anatomy and physiology, incorporating new genetic insights into the LGN’s cellular taxonomy, following the flow of information into LGN and out to the cortex, and, finally, reviewing the functional impact of attention. The final section summarizes the key points.
Lateral geniculate nucleus anatomy
The human LGN is an elongated, layered structure with the shape of a small bean ( Fig. 29.1A ) and a volume that can range from 77 to 152 cubic millimeters across individual subjects. All human LGNs have six main layers in the posterior half of the nucleus representing central vision and two layers in the smaller segment representing monocular peripheral vision ( Fig. 29.1B ). The six main layers of the LGN are classified by cell size as parvocellular (parvo: small in Latin) or magnocellular (magno: big in Latin), and they are separated by thin koniocellular layers with sparse small cells (konio: dust in Greek). The layers are numbered, from ventral to dorsal, as M1 to M2 for the magnocellular layers, P3 to P6 for the parvocellular layers, and K1 to K6 for the koniocellular layers ( Fig. 29.1C ).

Every LGN layer represents half of the contralateral visual field. The LGN layers of the left hemisphere represent the right visual hemifield and the layers of the right hemisphere the left visual hemifield. Because each visual hemifield is seen with the nasal retina from one eye and temporal retina from the other, the two eyes need to be represented in the same LGN. The representations of each eye are segregated into different LGN layers. The nasal retina from the contralateral eye is represented in layers M1, P4, P6, K1, ventral K2, K4, and K6, whereas the temporal retina from the ipsilateral eye is represented in layers M2, P3, P5, dorsal K2, K3, and K5 ( Fig. 29.1C ). The layers receiving contralateral input are the only ones representing the monocular peripheral region; therefore, those representing the ipsilateral eye are always shorter because they represent a smaller visual field.
The repeated representations of the visual hemifield in the different LGN layers are precisely aligned such that one point in visual space is represented along a line crossing all layers roughly perpendicularly. This retinotopic alignment is so precise that the blind spot caused by the optic nerve is represented by a gap of LGN tissue free of cells, which maintains the retinotopic alignment of the contralateral and ipsilateral layers (see Fig. 29.6 ). The laminar organization of the LGN is similar in humans and other primates such as macaques but differs across mammalian species. In spite of these variations, several organizing principles are evolutionarily preserved in the LGN, including the segregation of eye inputs and the basic neuronal circuitry.

Lateral geniculate nucleus development and cellular taxonomy
Work in mice shows that the LGN is generated alongside approximately 50 other thalamic nuclei from a small patch of neuroepithelium located in the embryonic diencephalon. Under the control of developmental programs, a small group of neural precursors bud off this patch to create the nascent LGN, differentiating in the womb to create excitatory neurons and glia. LGN is among the only dorsal thalamic nuclei possessing inhibitory interneurons, which in rodents are born in the ventral LGN and forebrain, and migrate into the LGN after the excitatory neurons are generated. Primate LGN development is likely to follow a similar plan, as recent comparative genetic analyses reveal that rodent, macaque, and human LGN neurons can be divided into transcriptomically similar subclasses.
Droplet sequencing methods allow investigators to acquire millions of individual neural transcriptomes from human, macaque, and mouse LGN, and then group neurons according to their gene expression ( Box 29.1 ). Comparing such transcriptomic cellular atlases across species reveals that primate magnocellular and parvocellular LGN cells selectively express the calcium buffer parvalbumin-1 (Pvalb1), whereas koniocellular LGN cells express the calcium buffer calbindin-1 (Calb1). Interestingly, this pair of genes labels largely exclusive subpopulations of excitatory neurons that reside in the core (Pvalb1) or shell (Calb1) regions of rodent LGN. Atlas comparisons also show a diversity of interneurons for each species and predict three or four different inhibitory types for primates occurring at an abundance of about one-quarter that of their excitatory counterparts. These new data confirm older evidence showing that magnocellular LGN cells selectively express genes such as neurofilament heavy chain and Robo2, whereas parvocellular LGN cells express the transcription factor Foxp2.
Neural circuits are viewed through the lens of their man-made electrical counterparts—the brain’s many individual circuits are thought to wire unique subsets of neural types together so they can perform computations. Thus, defining a full list of the brain’s neural types (parts list) has become a central goal in the neurosciences. A marriage of combinatorics, microfluidics, and deep sequencing has yielded a new technology to fuel this effort. This approach, called droplet sequencing, works as follows. (1) A brain or brain region is dissociated into a suspension of individual cells. (2) Each cell is then encapsulated in a droplet of oil using a microfluidic device that collides such cell-containing droplets with another droplet containing lysis buffer, an inert bead decorated with DNA primers, and reverse transcriptase. (3) Upon collision, cells release mRNAs that bind the bead-anchored primers and permit reverse transcriptase to copy their code into a DNA compliment (cDNA). Thus, each bead contains cDNA strands bearing a unique barcode, a communal bead barcode. (4) After all cells have been processed, the droplets are combined and broken open for a final round of amplification, and the entire library is subjected to deep sequencing. Comparing mRNA species bearing one bead barcode to another permits individual cells to be grouped into transcriptomic atlases that describe cell types. Each single-cell transcriptome costs fractions of a cent, making large-scale analysis of neural genomes relatively cheap—atlases now exist for every principal type in the retina, the lateral geniculate nucleus, and visual cortex of several species providing a tangible route to translate insights from experimental models, such as mouse, to man.

A notable omission from this effort is a lack of genetic analyses in cats, in which morphologic and functional characterization of LGN neural types is most complete. In this species, relay neurons are categorized into X-, Y-, and W-cells, which each possess a characteristic dendritic structure, laminar organization, and visual response. W-cells are believed to be analogous to koniocellular cells in primates and shell neurons in mouse. A well-accepted correspondence between the remaining LGN types in cats, primates, and mice does not exist currently. However, several functional studies suggest that magnocellular and parvocellular cells are composed of several subtypes, consistent with recent work in rodents showing 20 functionally distinct LGN neuron classes. A clearer picture of the cell-type diversity in the LGN is important to our understanding of how this structure combines inputs originating from retina, cortex, midbrain, and brainstem. We consider major sources of input in the next section.
Lateral geniculate nucleus inputs
The LGN is the main link between eye and cortex. Its excitatory neurons, called relay cells, integrate excitatory input from RGC axons and transfer this information to the cortex for further analysis. Only a relatively small percentage of the LGN synapses are made by RGC axons, whereas many others arise from axons originating in the visual cortex and brain stem. Thus, the LGN has been proposed to act as a “smart” filter that titers visual input reaching the V1 according to internal feedback. Next we briefly overview the main LGN inputs ( Fig. 29.2D ).

Retinal inputs
A family of RGC types decompose incoming visual stimuli. Some RGCs compare stimuli falling in their receptive field center with stimuli in their surround. ON RGCs fire when the stimulus luminance is higher at the center than surround, whereas OFF RGCs fire when the stimulus luminance is higher at the surround than the center. In humans, midget RGCs receive center input from a single cone. Parasol RGCs boast larger dendritic arbors that integrate from several cones, making their view of the world larger but devoid of color information. Yet other RGCs have been described in the peripheral parts of the primate retina that encode other features such as stimulus motion. These three RGC classes project to specific layers within the LGN, with midget RGCs providing input to the parvocellular layers, parasol RGCs to the magnocellular layers, and all other RGC types innervating the koniocellular layers ( Fig. 29.2A–C ). New genetic methods (see Box 29.1 ) have provided a nearly complete cellular atlas of primate RGCs and predict a total of 22 primate RGC types. As expected from previous work, midget and parasol types are the most abundant (~90% of all peripheral and foveal RGCs), but a sizable fraction of primate RGCs with unknown function remain. Such non-midget, non-parasol RGC types likely drive the koniocellular pathway and express genes that are also expressed by the approximately 50 feature-detecting RGCs types found in mice. Whether this transcriptomic similarity also reflects a functional similarity across species is not known, but the type-specific marker genes provide a clear way to address this idea. Indeed, early comparative studies appear to have found a direction-selective primate RGC type with morphology and function matching that seen in mice.
Cortical inputs
Nearly 30% of the LGN excitatory synapses form between relay cells and the axons of layer 6 (L6) pyramidal cells projecting from cortex. Such feedback connections are stream specific. For example, the magnocellular LGN layers receive input from a population of L6 cortical neurons that are anatomically and functionally distinct from those providing input to the parvocellular layers. Unlike their RGC counterparts, these cortical feedback axons connect only with relay cells and not with resident interneurons. L6 neurons form small boutons located on the distal dendrites of relay cells that cannot drive LGN spiking on their own. Instead, these inputs are thought to modulate ongoing relay neuron activity.
Inputs from the thalamic reticular nucleus
The antero-latero-dorsal surface of the entire thalamus is wrapped in a thin nucleus that exhibits a strong suppressive effect on every thalamic nucleus including the LGN. This shell, called the thalamic reticular nucleus (TRN), contains inhibitory neurons and has a loose topographic organization that matches the inputs it receives from sensory cortices, motor regions, and brainstem structures. Remarkably little is known about these neurons, but several recent genetic studies have identified a host of disease-relevant mutations that affect genes strongly expressed by the TRN. Visual TRN integrates descending input from L6 V1 neurons with ascending input from LGN relay cells to deliver feedback inhibition to LGN neurons.
Inputs from the brain stem
The LGN is a major target of neuromodulatory inputs emanating from diverse brain regions that include histaminergic inputs from the hypothalamus, serotonergic inputs from the dorsal raphe, cholinergic inputs from pontine nuclei, and noradrenergic inputs from the locus coeruleus. These inputs are believed to diffusely release their neuromodulators onto LGN neurons and activate G-protein–coupled receptors that hyperpolarize or depolarize the membrane potential of LGN relay neurons, LGN inhibitory neurons, and retinal inputs.
Inputs from the superior colliculus
Visual input from the eye is forked—RGC axons branch to innervate both the LGN and a midbrain sensorimotor area called the superior colliculus (SC). Inputs in this latter structure are organized retinotopically in the superficial-most layers of SC, which are registered to a trio of lower layers containing maps of auditory, somatosensory, and motor space. LGN is a major target of the SC. In primates, SC inputs target the koniocellular layers of the LGN and recent work in cats and mice suggest that such inputs can “drive” LGN neurons to fire action potentials. Thus, some LGN spikes sent to cortex appear to take a scenic route through the SC. This strong SC input remains poorly studied; however, the SC→LGN projection has been found in over 15 different mammalian species, suggesting a conserved evolutionary function. This function is likely related to motion processing, as most of the SC inputs target thalamic structures (koniocellular LGN layers, pulvinar) that project to the middle-temporal area (area MT).
Inputs from local interneurons
Local interneurons comprise approximately 25% to 30% of LGN neurons in macaques, approximately 20% to 25% in cats, and 10% to 20% in rats and mice. These neurons extend large dendritic arbors with short axons that ramify within the extent of their dendritic arbor. They integrate feedforward input from RGCs and from other LGN interneurons and use this output to inhibit relay neurons. Interneurons in the LGN are atypical—both axons and dendrites are capable of releasing neurotransmitter. Modeling studies indicate that dendritic release sites are electrically insulated from each other, and the axonal compartment isolated from the dendrites. In cats, dendritic synapses participate in triads that inhibit X-cells, whereas axonal synapses form preferentially on Y-cells. It is also worth noting that dendritic synapses are considerably more common than axonal synapses in cats. Whether these arrangements are present in other species is not clear.
Lateral geniculate nucleus circuitry
The remarkable diversity of inputs to the LGN runs at odds with the synapses they form in this structure—most connections can be easily categorized into three classes based on their ultrastructure. One class bears large terminals housing hundreds of round vesicles docked at multiple release sites (called RL), another has small terminals with round vesicles (RS), and the last has a flat nerve terminal with pleomorphic vesicles (F). Retinal axons form all RL terminals (~5–10% of LGN synapses), internal inputs from cortex and elsewhere form all RS terminals (~80% of LGN synapses), and inhibitory inputs form all F synapses (~15% of LGN synapses). Local interneuron dendrites form F1 synapses with high synaptic vesicle density and interneuron or reticular axons form F2 synapses with lower synaptic vesicle density. Next we highlight three commonly observed microcircuit arrangements and discuss their potential roles in LGN function.
Retinal ganglion cell synapses localize to the soma and proximal dendrites of LGN neurons
Synapses from RGCs account for only a small fraction of the total number of synapses with the LGN. However, these connections are by far the strongest. Their outsized influence on relay neuron firing arises in part from the unique, multivesicular RL morphology and their subcellular location on the proximal dendrites and somata of LGN neurons ( Fig. 29.3 ). This places their excitatory potentials close to the axon hillock where LGN neurons initiate action potentials. Convergence estimates in slice recordings from cat and rodent LGN suggest that an adult LGN neuron receives input from between one and four different retinal axons, with one RGC input providing most of the excitatory drive. The weaker RGC inputs are thought to synapse on the distal dendrites of an LGN neuron’s arbor and often show a more RS-like appearance. Thus, an LGN neuron’s view of the world is shaped predominantly by a small number of RGCs.

Retinal and local interneuron synapses are arranged as triads
The RL terminals of retinal axons often synapse with the dendrites of the LGN relay cell dendrites and those of the relay cell’s presynaptic interneuron. This unit, termed a triad , positions feedforward excitatory and inhibitory synapses within a few microns of one another and often encapsulates these two structures within a glial sheath. Triads impose an exacting form of feedforward inhibition upon RL terminal activation because inhibition from adjacent F terminals would shunt excitatory drive within milliseconds. This arrangement is thought to favor retinal inputs that fire in tight synchrony, as lagging or leading inputs are likely to be silenced by local inhibition. Whether such synchrony is used to encode different features of a stimulus is currently unknown.
Drivers and modulators
Although the exact significance of the RL, RS, and F stereotypy is not clear, the consistency of these morphologic definitions across the LGN of rodents, cats, primates, and humans indicates a conserved computational role in the LGN. A prominent view of LGN function posits that inputs bearing RL versus RS terminals can be defined as being drivers or modulators, respectively. In this scheme, only RL inputs can evoke spikes from LGN relay cells; RS inputs modulate resting potential and therefore the burst versus tonic firing status of the relay cell. There is significant morphologic and ex vivo functional data in support of this hypotheses, but in vivo evidence is lacking. Although a large body of in vivo work is consistent with the notion that feedback inputs (bearing RS terminals) cannot evoke action potentials in LGN neurons, many of these studies were performed under anesthesia, which is now known to exert a powerful suppression of geniculate circuitry. Repeating these studies, perhaps with new optogenetic tools, would offer a way to resolve this issue.
Lateral geniculate nucleus outputs
The main output of the LGN goes to the primary visual cortex and is essential for the emergence of conscious visual perception. In macaques, the axons from magnocellular, parvocellular, and koniocellular LGN neurons are vertically segregated in different cortical layers ( Fig. 29.4A ). The LGN axons are densest in layer 4Cα for magnocellular neurons, 4Cβ for parvocellular neurons, and layers 4 A and 3 for koniocellular neurons. The LGN axons are also horizontally segregated. The axons from the contralateral and ipsilateral eyes segregate horizontally in different ocular dominance columns and the LGN axons from koniocellular neurons also segregate horizontally in patches of cells with high cytochrome oxidase activity known as blobs. Such blobs correlate with dense thalamic innervation and are believed to reflect the energy demands of high firing rates in geniculocortical transmission.

The synaptic boutons made by LGN axons are large, are located proximally in the dendrites of cortical neurons, make multiple synaptic contacts with each cortical target, and have multiple mitochondria to maintain high metabolic activity ( Fig. 29.4B ). Consistent with the large size of the synaptic boutons, the excitatory postsynaptic currents generated by single geniculocortical axons are strong ( Fig. 29.4C ) and show robust synaptic depression. Moreover, the arrival of a spike through a single geniculocortical axon is strongly correlated with a rapid increase in the spiking activity of the cortical targets ( Fig. 29.4D ), which are tightly restricted to the cortical layer targeted by the axon ( Fig. 29.4E ). As expected from a strong monosynaptic connection, the receptive field properties of each geniculate neuron and its cortical targets are precisely matched in spatial position, ocular dominance, and ON-OFF receptive field polarity ( Fig. 29.4D ). The number of LGN neurons varies greatly across species and individuals of the same species, but is strongly correlated with the volume of primary visual cortex and the cortical resources available to process visual information.
In addition to the primary visual cortex, the LGN also projects to the visual sector of the TRN, a nucleus with inhibitory neurons that regulate the LGN output through feedforward inhibition. The LGN also projects to several extrastriate visual cortical areas such as area V2 and the area MT. Specifically, the LGN projection to area MT originates in a small number of koniocellular LGN cells that do not project to primary visual cortex and may mediate the residual vision referred to as “blindsight” in patients with large lesions in primary visual cortex ( Box 29.2 ).
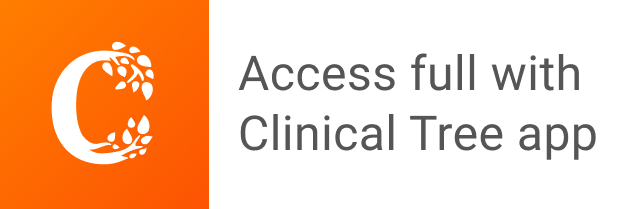